Recent Advances in Understanding the Geology of Diamonds
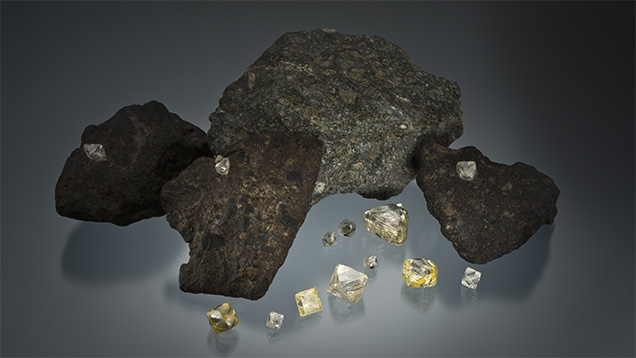
ABSTRACT
It has been more than two decades since diamond ages have proven to be up to billions of years older than their host magmas of kimberlite or lamproite. Since then, there have been significant advances in the analysis of diamonds and their mineral inclusions, in the understanding of diamond-forming fluids in the mantle, and in the relationship of diamonds to the deep geology of the continents and the convecting mantle. The occurrence of natural diamonds is remarkable and important to earth studies. This article reviews current thinking of where, how, when, and why natural diamonds form.
INTRODUCTION
Research into natural diamonds (figure 1) has emerged over the last two decades as one of the keys to understanding the deep earth. Analytical advances, improved geologic knowledge, and the emergence of new diamond-producing regions (such as the Slave craton of Canada) have all contributed to this change. The most prized specimens for research are flawed with visible inclusions (figure 2), for these carry actual samples of mantle minerals from depths as great as 800 km beneath the surface. Diamond provides the perfect container for mantle minerals, isolating them from the high pressure and temperature reactions within the earth for geologic time scales. Even low elemental concentrations and minute features in diamond can now be analyzed using instruments with higher sensitivity and resolution. As a result, study combining the inclusion and its diamond host is a powerful tool for geologic research, which itself has improved our understanding of diamond formation.
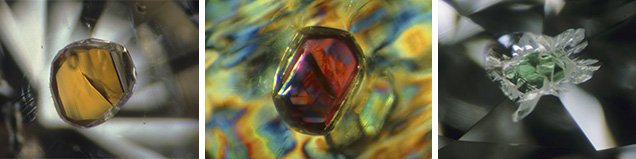
The purpose of this article is to describe our current understanding of where, how, when, and why natural diamonds have been formed. This article reviews currently accepted areas of knowledge, along with topics that are still the subject of ongoing research, where science does not yet have all the answers. This basic subject was discussed more than two decades ago in Gems & Gemology (Kirkley et al., 1991); since then, there have been major advances in our understanding of diamond geology. Recent work of the research community (summarized in Pearson and Shirey, 1999; Cartigny, 2005; Harlow and Davies, 2005; Stachel et al., 2005, 2009; Stachel and Harris, 2008, 2009; Gurney et al., 2010; Shirey et al., 2013) has been of considerable interest to economic geologists searching for natural diamonds, guiding their models of how to explore for new occurrences. But it is also of importance to the practicing gemologist, since these are fundamental questions that a wearer of a beautiful diamond might ask. It is hoped that this article will give the gemologist a ready way to convey how nature first created the rough diamonds.
For the reader who is unfamiliar with geologic terms, a glossary is presented at the end of the article. Terms listed in the glossary are italicized on their first use in the text.
GEOLOGY AND THE DISTRIBUTION OF DIAMONDS ON EARTH
Carbon Abundance. Carbon is widely dissolved in the earth’s silicate minerals at part-per-million levels and lower. But whenever carbon occurs as a free species, diamonds have the potential to form. Carbon in the earth can occur in oxidized forms, such as when bound with oxygen in CO2 or CO3, or in reduced forms such as diamond, graphite, or bound with hydrogen in methane and other organic molecules. The experimentally determined pressure-temperature conditions where diamond is stable (figure 3) dictate formation pressures higher than 40 thousand atmospheres (4 GPa) and temperatures of 950–1400ºC. While these pressure-temperature conditions seem extreme, for a large rocky planet such as ours, they are not. Within the earth, temperature always rises with depth along a path known as the geothermal gradient, which is typically high enough for diamond growth at the necessary pressures. Thus, diamond can potentially form in any region of the earth where the depth of the crust or the mantle provides high enough pressure, because the temperature will also be high enough.
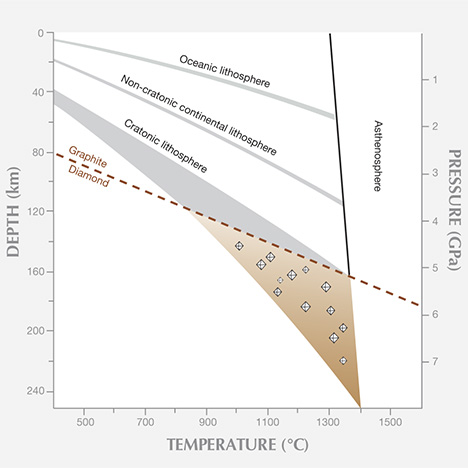
Most of the mantle is within the field of diamond stability. The crust, which is normally too thin (usually less than 40 km thick) to lie within this field, can do so only if it has been thickened by the geologic processes related to plate tectonics. Yet diamonds are very rare because the mantle has a relatively low abundance of carbon. Furthermore, diamonds are far from evenly distributed throughout the earth—they are found in mineable quantities only in very unique geologic settings. Why is this so?
Geologic Age of Continental Rocks. Earth is special among the planets in that it has two crustal types, continental and oceanic, that sit at two very different heights, approximately 840 meters above and 3,840 meters below sea level on average. This happens because the continental crust contains more of the lighter elements such as silicon and aluminum, and is underlain by a thickened mantle keel (described below), while the oceanic crust is composed of heavier elements such as iron and calcium and is not underlain by a thickened mantle keel. The continental crust is old—up to four billion years old. Its oldest parts, the ancient continental nuclei, or cratons, are isolated in the interior of the continent by belts of successively younger continental crust (figure 4). By comparison, oceanic crust is much younger and progresses regularly in age from zero (formation today) to the oldest known ocean floor, which is about 0.2 billion years old. This basic age distribution of rocks at the earth’s surface (Hurley and Rand, 1969) became widely known within five years of the acceptance of plate tectonics theory in the mid-1960s, as naturally decaying radioactive elements (uranium, thorium, and rubidium) provided a quantitative way to measure the geologic age of exposed crustal rocks.
Plate Tectonics and Diamonds. Plate tectonics is the modern unifying theory that explains the earth’s active geologic processes today, and is thought to have operated perhaps for as long as the latter half of the planet’s history. No other planets in the solar system apparently have plate tectonics. The earth’s upper surface is composed of rigid, lithospheric plates of crustal rock (too stiff to flow on geologic time scales, yet stiff enough to break and cause earthquakes) underlain by mantle rock. Surface deformation, volcanic activity, and earthquakes occur more readily at the margins of plates than at their interior. Two general types of lithosphere can occur on the same plate: continental and oceanic. Continental lithosphere is thickest where it is oldest. It can be more than twice the thickness of oceanic lithosphere, which is geologically younger. The latter is constantly being created at mid-ocean ridges where seafloor spreading occurs, and where oceanic crust is recycled back into the mantle by the process of subduction. The movement of the plates occurs on the mobile portion of the mantle known as the asthenosphere, and it is driven by deeper flow of the mantle, a process known as convection. The power for convection comes from the sinking of the oceanic lithospheric plates, the heat generated in the mantle by radioactive decay, and the return flow of warmer mantle. Plate tectonics is critical to diamond formation in two ways: It permits the recycling of surficial carbon, and it enables mantle melting, both of which allow the creation of diamond-forming fluids/melts.
Continental regions that long ago ceased participating in active plate tectonic processes such as rifting, mountain building, or subduction are known as continental cratons. They are easily defined by an absence of earthquake activity. Such regions have been leveled by long-term weathering and erosion, though they may be relatively recently uplifted, as is the case for southern Africa. Most continents contain several cratons (again, see figure 4) joined by younger crust at times long after their creation. A craton always contains the oldest rocks within its host continent, and it typically has ages older than 2.5 billion years, from a geologic era known as the Archean. Common usage has evolved so that the term craton often implies the Archean portion. But strictly speaking, cratons are not limited to the Archean era. Numerous younger terranes (e.g., 1 billion to 2.5 billion years old) now fit the requirement for long-term geologic stability. Thus, the plate tectonic process of continental assembly and breakup has led these younger terranes to be attached to the older cratonic core, so that they too can effectively be considered part of the craton. Both the Slave craton in Canada and the Kaapvaal craton in southern Africa are the Archean components of the larger Laurentia and Kalahari supercratons, respectively.
For economic geologists, the most important point is the striking correspondence between the oldest Archean portion of a craton and diamond occurrences, especially those hosted in kimberlite, the main carrier and hence “ore” of gem-quality diamond. This relationship, first formalized by Clifford (1966) and known as Clifford’s Rule, implies that the diamondiferous kimberlites erupted through the oldest Archean portions of the cratons, whereas non-diamondiferous kimberlites erupted through younger rocks. This relationship is nowhere more evident than the Kaapvaal craton, where all of the diamondiferous kimberlites are “on-craton” and all of the “off-craton” kimberlites are diamond-free. We will examine the geologic explanation for this below.
The erosion of ancient cratons has led to the weathering of surface exposures of kimberlite, and the release of diamonds to the regolith. Without crustal uplift, these diamonds remain trapped in geologic basins as in West Africa, Zimbabwe, and Brazil, where they can be panned for like gold. Where the craton has been uplifted, diamonds released from their host rocks have been transported by rivers (such as the Orange River in South Africa) and by longshore currents (such as the Benguela, along the continental shelf of the southern Atlantic Ocean). These alluvial diamonds are recovered by placer and marine mining techniques that are very different from hard-rock kimberlite mining. Although these specimens are found “off-craton,” they derive from “on-craton” kimberlites and are thus formed by those processes.
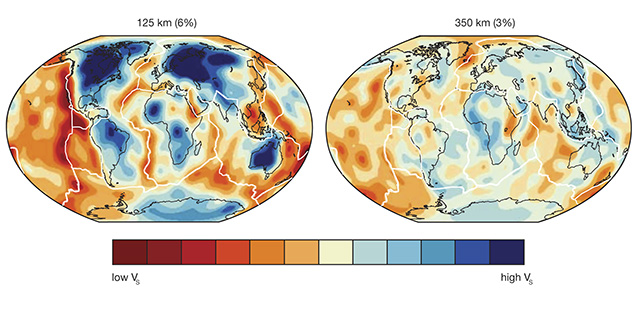
Mantle Keels Under Continental Cratons. Sitting beneath both oceanic and continental crust is rigid peridotitic mantle that, with the overlying crust, comprises the lithosphere. This region of rigid mantle is known to exist because it can rupture to cause earthquakes; the resultant seismic waves travel faster through it than the convecting but still solid mantle just below. Beneath the cratons, the lithospheric mantle extends from about 40 km depth down to perhaps 250–300 km (figures 5 and 6). Under the oceans, it only extends down to about 110 km (Jordan, 1979). Because of its downward-protruding shape and its long-term attachment to the continental crust of the craton, this portion of mantle has taken the term mantle keel. The mantle keel is a major reason for some features we associate with continents: tectonic stability, elevation above the ocean floor, and the occurrence of diamonds. The kimberlite eruptions that transport diamonds to the surface also carry samples of lithospheric mantle rocks called xenoliths. From these samples, we know much about the mantle keel beneath the continents, such as the fact that it also contains about 5% of the high-pressure form of basalt known as eclogite. The mantle keel hosts nearly all of the world’s gem diamonds, and thus it deserves more than passing attention when considering the geologic origin of diamonds.
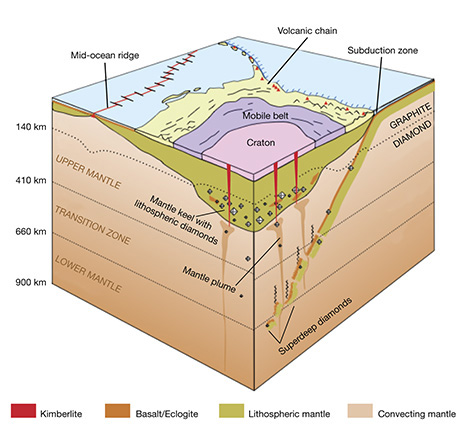
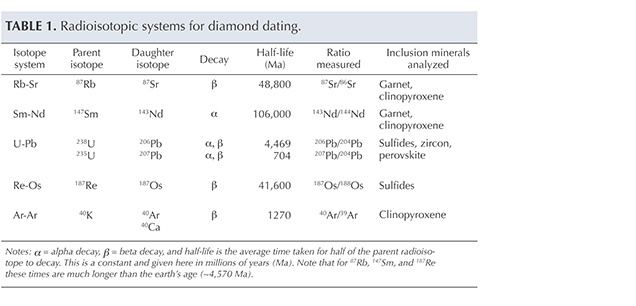
Naturally occurring radioactive elements present in small amounts in peridotite and eclogite allow geologists to measure the age of rock samples of the mantle keel (table 1). These include rhenium (Re), which decays over millions of years to osmium (Os), and samarium (Sm), which decays to neodymium (Nd). The age of the keel is the same (within uncertainties) as the geologic age of the overlying crust. Consequently, most geologists think that the crust and mantle keel of the continent were created together in a process of crust creation and craton stabilization. The duration of this process is poorly known, but may have taken many tens of millions of y ears, starting with the formation of the oldest continental crust (nearly four billion years ago). The significance of this for diamond formation is that the bottom 100 km of the mantle keel under each old continental crustal region is at high enough pressure and comparatively low temperature to allow diamonds to crystallize whenever they receive fluids saturated in carbon from the underlying convecting mantle. Thus, the keel bottom can be viewed as an “ice box” (albeit with higher temperatures), available for billions of years to store diamonds and keep them from entering mantle circulation, yet ready to be sampled by a rising kimberlite magma. Both peridotite and eclogite contain diamonds, but intact peridotites erupted to the surface with their diamonds in place are rare, while eclogites with their diamonds in place are common. (For a detailed description of rock type, see Kirkley et al., 1991.) The mineralogical reason for this is a result of the way diamonds crystallize within the keel itself.
Diamonds in Tectonically Active Areas. Clifford’s Rule demonstrates the connection of the ancient stable mantle keel to gem-quality diamonds. Its corollary is that geologic activity related to plate tectonics such as volcanism, mountain-building, and intrusive magmatism near the earth’s surface typically destroys diamonds, because it occurs at pressures, temperatures, or oxidizing conditions where diamond cannot crystallize or remain stable. The typical slow mantle upwelling and resultant magmatism that occur beneath an ocean island such as Hawaii offers a good example. Nonetheless, a few exceptions to Clifford’s Rule do exist, where diamonds are found in non-kimberlitic rocks formed in tectonic areas that were once active.
A few localities are known where a non-kimberlitic, subduction-related magma type carries diamonds: young microdiamonds in the Japan island arc (Mizukami et al., 2008), and 2.7 billion-year-old macrodiamonds in the Wawa belt of the Superior geologic province of Canada (Stachel et al., 2006). In both cases, diamonds appear to have been created not by direct subduction magmas themselves, but rather by late-stage magmas that produced a rock called a lamprophyre. These magmas were intruded as dikes and transported diamonds to the surface that may have been created elsewhere in the mantle. At the world’s largest diamond producer by carat weight, Australia’s Argyle mine (Shigley et al., 2001), and its smaller and younger cousin, the nearby Ellendale mine, specimens were brought to the surface by another non-kimberlitic magma that produced a rock called lamproite. These diamonds are about 1.5 billion years old. The Australian geologic provinces in which they occur are known as mobile belts, which also contain metamorphic crustal rocks that are slightly older (1.8 billion years old). The thermal pulse revealed by the study of crustal rocks at the surface is thought to result from a tectonic process that heated and recrystallized older lithospheric mantle while permitting the formation of diamonds at the same time (Smit et al., 2010).
More abundant than these examples are unique blocks of crustal rock known as ultra-high-pressure (UHP) metamorphic terranes. These were buried deep enough by crustal thickening, a process where portions of the crust override other portions and allow microdiamonds to crystallize, evenly distributed, throughout the buried crustal host rock with no apparent magmatic transport. Examples of this geologic setting occur in China, Germany, Norway, Russia, and Indonesia.
Perhaps the most prevalent example of diamonds formed by active geologic processes are those known as superdeep diamonds. These are usually not gem-quality macrodiamonds. Superdeep diamonds formed at great depths in freely convecting mantle beneath the continental lithosphere (again, see figure 6). They were brought up by the same kimberlitic volcanism that carried lithospheric diamonds, and therefore they are found in the same deposits. The high-pressure minerals they include show that they formed far below the 300 km approximate depths of kimberlite generation, perhaps as deep as 400–800 km (Harte, 2010). Thus, they must be carried into the depth of kimberlite generation by upwelling mantle convection in mantle plumes. Mantle plumes are thought to trigger kimberlite formation, because they bring hotter mantle to shallower depths where it begins to melt. Kimberlites form at the very first stages of mantle melting.
These examples of diamonds formed in actively convecting mantle are often subeconomic, lacking sufficient gem-quality stones. But they hold great scientific worth by preserving the record of dynamic geologic processes in the deep earth.
EMPLACEMENT AND HOST ROCKS OF DIAMONDS
The kimberlitic volcanism that carried diamonds to the earth’s surface is unique and rare; in fact, no kimberlite eruption has ever been witnessed. The association of diamonds with ancient cratons makes it clear that kimberlitic volcanism occurs exclusively in these stable continental crustal environments. Because kimberlites are unknown in the oceanic mantle, the presence of mantle keels under the continents and the mechanical impediment provided by the rigid keel appears to be an important aspect of kimberlitic magma formation. The stiff lithosphere slows the rise of upwelling mantle beneath it, at about the same depth where carbonate-bearing peridotite has been shown to begin to melt (figure 7). As melting begins, the first melts form in between the stiffer main silicate grains. These volatile-rich melts migrate rapidly through the silicate grains, separating a volatile-enriched kimberlite. Such an effect is missing under the oceans.
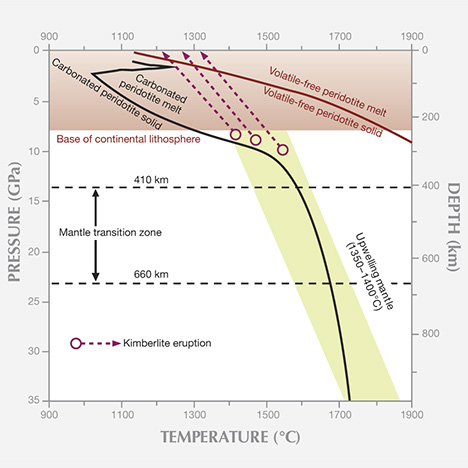
Magmas That Carry Diamonds. Diamonds are known to be carried to the earth’s surface in only three rare types of magmas: kimberlite, lamproite, and lamprophyre. Of the three types, kimberlites are by far the most important, with several hundred diamondiferous kimberlites known. Although the number of diamondiferous lamproites is much smaller, they do host the Argyle mine and notable subeconomic occurrences in the United States, India, and Australia. Lamprophyres are rarely diamondiferous; they are only of petrological interest as hosting the world’s oldest erupted diamonds, which occur at Wawa in Ontario, Canada. In general, all three magma types are: (1) derived by small amounts of melting deep within the mantle; (2) relatively high in volatile (H2O, CO2, F, or Cl) contents; (3) MgO-rich; (4) marked by rapid eruption; and (5) less oxidizing than more common basaltic magma. These features work together to transport diamond crystals upward to the surface without enough resorption to dissolve them (figure 8)—something that is just not possible with other melts from the mantle, such as the far more abundant basalt and its alkalic varieties.
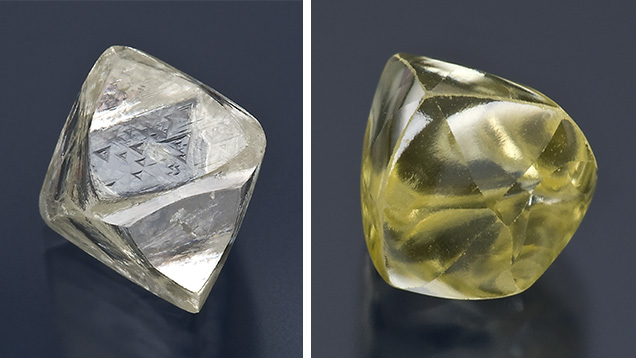
The classification of volcanic rocks by their textures, mineralogy, and chemical composition in a way that accounts for their genesis (Woolley et al., 1996) has historically been an inexact science. But such classification (table 2) is critical to finding and recognizing diamond-hosting rocks in the field—the essential first step to extracting gem diamonds. Kimberlites weather rapidly in the geologic sense, often forming exposures of low topographic relief, and even lakes. When exposed and fresh, they are dark bluish green to greenish gray rocks that rapidly turn brown and crumbly. Texturally, they are full of mineral grains and rock clasts ranging from the size of a watermelon down to small grain sizes that dominate the matrix (figure 9). These diamond-bearing rocks are distinguished from the related carbonatites by having an igneous carbonate mineral abundance of less than 50%. Experiments show that kimberlites and carbonatites can form a continuum in which carbonatites may beget kimberlites. Furthermore, carbonatites may be a ready source of diamond-forming fluids (e.g., Walter et al., 2008). But at the earth’s surface, carbonatites are almost never diamond-bearing. The simple reason is that their carbon is locked up in the carbonate mineral calcite (CaCO3), which simply has too much oxygen to allow carbon to exist in the elemental form needed to stabilize diamond.
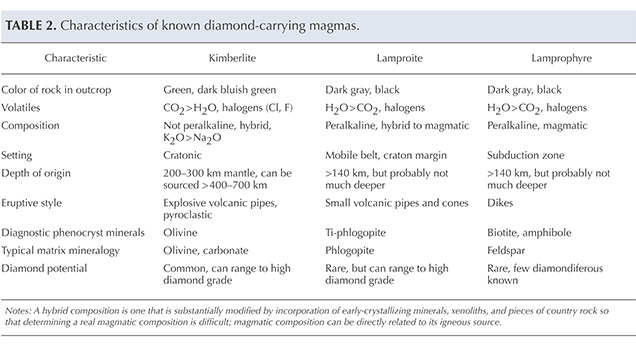
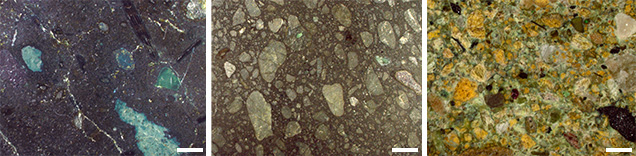
These three diamond-carrying rocks all lack the minerals melilite (Ca-Na-Mg-Al-silicate) and kalsilite (KAlSiO4), whose presence would indicate a different clan of rocks (Woolley et al., 1996) that are never diamond-bearing. If the magma forms a minor-size intrusion, is not peralkaline (Na2O+K2O greater than Al2O3), and is dominated by ferro-magnesian (mafic) mineral phenocrysts—more of which are olivine compared to lesser phenocrysts of magnesite, phlogopite, carbonate, or diopside—then the rock is a kimberlite (table 2). If these lesser phenocrysts dominate and the rock is peralkaline and contains obvious Ti-phlogopite, then it is a lamproite. If the rock is similar to a lamproite in being peralkaline but contains abundant biotite or amphibole, then it can be considered a lamprophyre. If this sounds confusing, you are not alone; many papers (summarized in Woolley et al., 1996) have been written to ensure that geologists who study diamondiferous rocks use consistent criteria in the field and laboratory. The point is that volcanic rocks that carry diamonds have a specific chemical and mineral composition, and can be recognized in outcrop or drill core by their textural characteristics above (again, see figure 9). Recognizing kimberlite in the field is important because diamonds are always so scarce in kimberlite that they are never visible in outcrop. Starting with the right rock is the first step to finding diamonds.
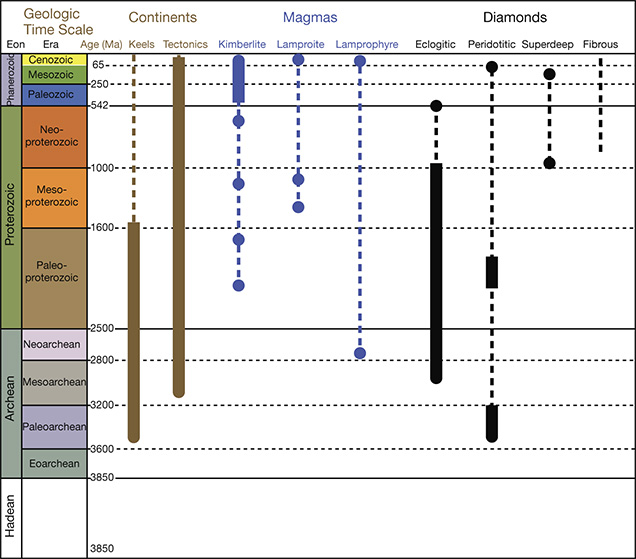
Typically, the host rocks that carry diamonds are younger than the diamonds and the ancient continental cratons they intrude, as shown in figure 10. With only a few exceptions (Argyle, Premier, and Wawa), all known diamond-bearing kimberlites are less than about 550 Ma (million years old) and most of them less than 300 Ma, with abundant episodes of kimberlite eruption at less than 120 Ma in southern Africa and less than 80 Ma in North America. Kimberlites are very quickly weathered and eroded rocks, so quickly—years, in fact—that this degradation cannot explain the preponderance of young kimberlitic volcanism. Instead there are likely some unique changes in mantle volatiles and the relationship of plate tectonic subduction to the mantle keels beneath cratons that account for this observation, but its explanation remains unknown.
How Emplacement Occurs. Kimberlites are difficult to interpret texturally, mineralogically, and chemically because of the high-energy mechanism of emplacement that breaks up the abundant extraneous materials they contain. Kimberlites are chaotic mixtures of xenoliths of crustal rocks and mantle, minerals released from the xenolith crumbling during eruption, phenocryst minerals, alteration minerals of these previous phases such as serpentine, and pieces of preexisting kimberlite. The texture of the host kimberlite and the relative proportions of these components (including diamonds) vary greatly with depth in the kimberlite pipe. The rock is a mixture consisting of preexisting materials and those that crystallized during the eruption. Geologists describe this as a hybrid rock (figure 11), which they do not consider a true representation of melt composition. Typical kimberlites and lamproites are hybrid rocks, and lamprophyres less so even though they may contain abundant xenoliths.
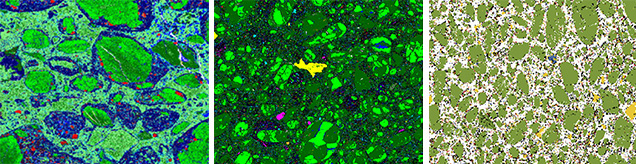
The volcanic emplacement of a kimberlite, though it has never actually been witnessed, is thought to be one of this planet’s most dynamic volcanic processes. Kimberlites propagate upward through the lithosphere by hydraulically fracturing the overlying rock. They move at relatively high velocity for a magma (from 4 to 20 meters per second; Sparks et al., 2006), and the progressively lower pressure as they rise allows a vapor phase to exsolve from the system. This vapor phase keeps the components fluidized, and it supports a column of entrained material that becomes rounded and broken during transport. The evolution of the magma from its deep mantle source is a complex history of changing features such as the magma composition (siliceous or carbonaceous), the proportion of the system that is condensed (magma+rock and mineral fragments) versus gaseous (H2O+CO2), and the ratio of CO2 to H2O.
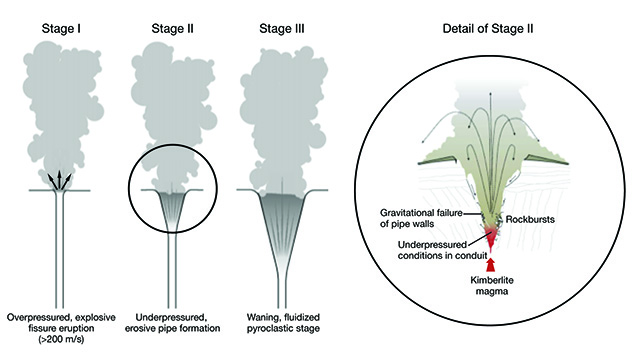
A kimberlite magma can start at depths as great as 200–300 km, but must be generated at least below the depths where diamonds are stable (greater than 140 km) in order to pick them up from their lithospheric source. The source of kimberlite, either within or below the lithosphere, is a matter of active debate in the geologic co mmunity. Theory and field observation led Sparks et al. (2006) to propose a four-stage model of kimberlite eruption (figure 12):
- Stage I: Overpressured, explosive fissure eruption producing high vent velocities (greater than 200 m/s)
- Stage II: Underpressured, erosive pipe formation causing brecciation near the surface
- Stage III: Waning, fluidized pyroclastic stage producing volcaniclastic kimberlite ranging from massive to layered
- Stage IV: Post-eruptive hydrothermal metamorphism producing widespread serpentinization and crater fill
Fallback of volcanic material can occur once the eruption halts, but the kimberlitic rock formed during the latter three eruptive stages is typically well-preserved in the kimberlite pipe (figure 13). Their field interpretation by volcanologists, aside from being pivotal to understanding the nature of these eruptions, forms the basis for determining the distribution of diamonds in the kimberlite. This distribution is the essential step to evaluating the diamond grade of any kimberlite. Establishing diamond grade is a laborious process that combines bulk assay for diamonds in large amounts of exposed kimberlite (in some cases hundreds of tons) with core drilling of the unexposed kimberlite. The goal of this process is to accurately estimate the size of the kimberlite that could be mined (figure 14), the carats of diamond per hundred tons of rock, and the revenue per ton versus mining cost per ton.
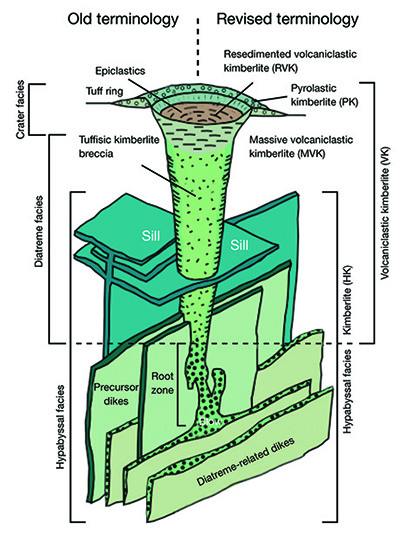
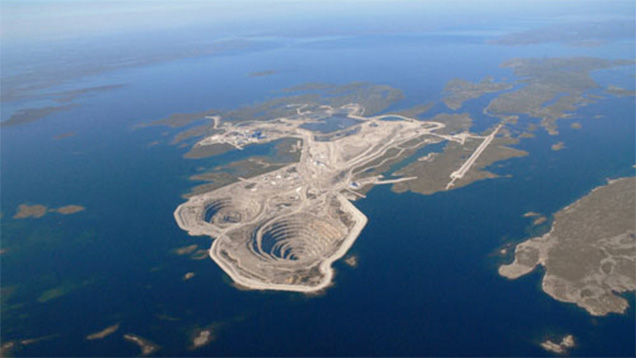
The high-energy dynamics and changing conditions of a kimberlite eruption leave their textural imprint on the minerals and rock fragments carried by a kimberlitic magma. From these mineral clues and the textures described above, the geologist must deduce the nature of the eruption. Foreign rocks (xenoliths) of upper crust such as shale, dolomite, and basalt, or of lower crust such as felsic and mafic granulite, are commonly found in the kimberlite pipe. Common too are xenoliths of mantle rocks such as harzburgite, lherzolite, websterite, and eclogite—the more fragile of which (e.g., magnesite-bearing harzburgite) are easier to break apart into their constituent minerals.
These foreign minerals, or xenocrysts, include olivine, clinopyroxene, orthopyroxene, garnet, ilmenite, and diamond itself, whose composition and relative abundance can reveal the makeup of the lithospheric mantle even in the absence of discrete rocks. As we will see below, such xenocrysts become the main prospecting tool for finding new diamondiferous kimberlites. Crystallizing directly out of the kimberlitic magma are phenocrysts of olivine, zircon, phlogopite, and groundmass perovskite. The latter three minerals can be used to determine the absolute geologic age and source composition of the kimberlite.
Very rarely, diamond can precipitate from the kimberlite. When it does, it can occur as overgrowths on monocrystalline diamond cores, as xenocrystic diamond hosts, and as microdiamonds. Any of these minerals, once formed or liberated, is subject to modification in the dynamic kimberlite eruption. Breakage, abrasion, and resorption may occur. Perhaps the most dramatic examples of resorption come from diamonds themselves (again, see figure 8), which display morphologies that range from simple etching to even teardrop shapes. There also is a noticeable difference between the perfect crystal morphology of type I diamonds and the cleavage fragments often seen in type II specimens.
Finding New Diamonds. Until the early 20th century, diamonds—even the famed Koh-i-Noor, Hope, and Cullinan—were typically found in alluvial or surface deposits, more or less by accident. The richness of the alluvial deposits of the Vaal and Orange Rivers of South Africa eventually led to the discovery of kimberlite and the famous workings around Kimberley, establishing kimberlite as the primary volcanic host of diamonds. From that point, exploration techniques centered on the best ways to find diamondiferous kimberlite using modern scientific methods. To the list of early alluvial diamond producers (e.g., South Africa, Namibia, India, Congo, and Brazil) have been added other hard ground or primary rock countries (namely Botswana, Russia, Australia, and Canada), which have greatly increased worldwide production.
As with other valuable ores, diamond exploration has become increasingly sophisticated and now includes some combination of different methods: geophysical techniques (airborne magnetic surveying, electrical resistivity, and gravity); geologic modeling (isotopic dating of ancient terranes and recognition of the history of their geologic modification); mineral analysis (garnet, ilmenite, and spinel indicator minerals); and geochemistry (surficial materials). Geophysical techniques and geologic modeling are useful for general narrowing of the exploration target area on a continental scale, whereas mineral analysis and geochemistry are applied on the ground when relatively close to the kimberlite.
Continental cratons are the first target for diamondiferous kimberlite exploration (see “Geology and the Distribution of Diamonds on Earth” and Clifford’s Rule above). By definition, these regions are not orogenically active. They consist of flat or peneplaned surfaces that can be deeply weathered (Brazil and Australia), covered by desert sands (Botswana), uplifted and eroded (South Africa), or extensively glaciated (Russia and Canada). Locating diamondiferous kimberlites is challenging due to their small surface outcrop and their tendency to weather faster than the surrounding crystalline country rock, which means the pipes are often hidden beneath vegetation, unconsolidated surface deposits, or lakes (figure 15).
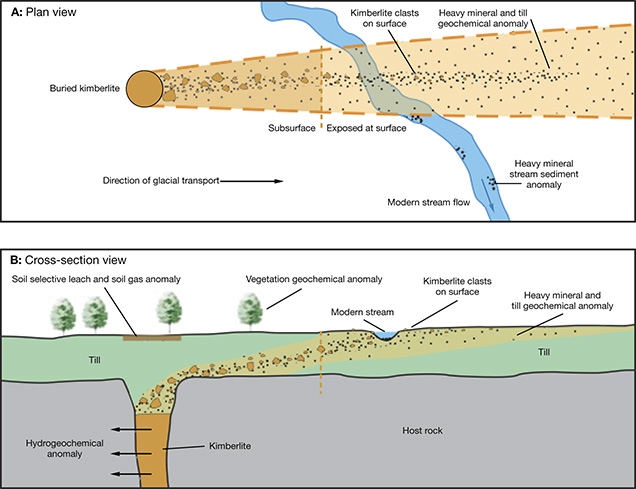
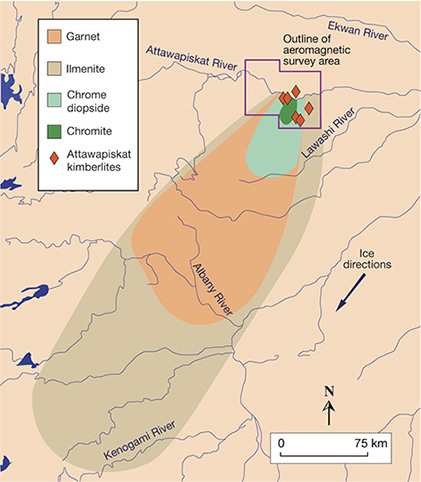
One very successful method for locating diamondiferous kimberlites employs a search in surficial deposits for actual fragments of the kimberlite, or for grain-sized indicator minerals weathered from these fragments (figure 16). These grains survive erosion, and their presence in sediments and soils is a predictor of whether a nearby kimberlite might contain diamonds. Indicator minerals range from single grains of silicate and oxide phases that have been released from mantle xenoliths broken up during sampling and transport by the kimberlite, to actual phenocryst phases in the kimberlite (Cookenboo and Grütter, 2010). In kimberlite, indicator minerals (figure 17) are much more abundant than diamonds, serving as markers for the chemically depleted mantle that can contain diamonds at depth. Furthermore, systematic mapping of the pattern of indicator minerals in surface deposits such as till, glaciofluvial sediments, or beach and stream sediments can point to the location of a kimberlite (McClenaghan and Kjarsgaard, 2007).
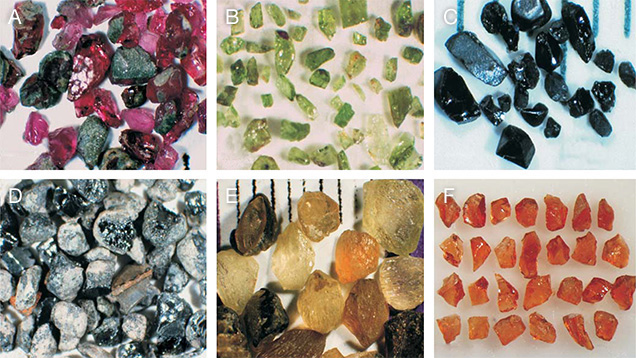
The key feature of the indicator mineral is its chemical composition as analyzed with the electron microprobe. A mineral is an indicator when its composition is characteristic of either the extreme melt depletion that typifies Archean continental mantle keels (peridotitic diamonds), or the high pressures found in subducted basaltic slabs (eclogitic diamonds). Indicators for peridotitic diamonds are so-called G10 pyrope garnets (high Cr and low Ca), chromite (high Cr + Mg and low Al + Ti), diopside (high Cr + Al), and orthopyroxene (high Mg/Mg+Fe). For eclogitic diamonds, Cr-poor garnets (high Na + Ti) and diopside (high Na) are commonly used. Mg-rich ilmenite (picroilmenite) is a general kimberlite indicator. The relationship between indicator mineral composition and diamond can be imperfect, and a more sophisticated approach uses pressure and temperature relations (geothermobarometers) deduced from the composition of individual minerals as if they were in equilibrium with other coexisting mantle minerals (Cookenboo and Grütter, 2010). This approach can be applied to a wider range of mineral compositions, and it has provided more sensitivity in locating mantle lithosphere capable of hosting diamond.
Exploration tools based on indicator minerals and surficial geochemistry are tailored to the nature of the weathering process. In deeply weathered and arid climates, vegetation, termite mounds, and geochemical anomalies directly overlie kimberlites and streambeds that potentially hold diamond placer deposits. In heavily glaciated terranes, kimberlite indicators are dispersed for tens of kilometers in patterns that relate to ice-flow directions (figure 16; Kjarsgaard and Levinson, 2002). In glaciated settings, other indicators include geochemical analysis of till, soil, soil gas, biota, and groundwater (figure 15; McClenaghan and Kjarsgaard, 2007). Under these conditions, regional surveys have proven quite effective in locating kimberlitic targets.
The success of these exploration methods has led directly to the discovery of some of the world’s most productive diamond mines, including Orapa and Jwaneng (Botswana’s Zimbabwe/Kaapvaal craton) and Ekati and Diavik (Canada’s Slave craton). As with all mined commodities, diamond exploration is highly dependent on price, global economic cycles, and rapidly changing mining company partnerships (to fund the costly exploration techniques). This search effort goes through boom and bust periods. Insightful case-study descriptions can be found on the Argyle deposit (Shigley et al., 2001) and occurrences in Canada (Kjarsgaard and Levinson, 2002).
The Argyle case study provides a perfect example of how long-known but sparse alluvial diamond occurrences in an arid and unglaciated terrain were combined with unconventional thinking, perseverance, and scientific methods to bring about the world’s most productive deposit. Of all the aspects of the Argyle discovery, perhaps the most important is unconventional thinking, because the diamonds occur in lamproite (all other rich deposits are in kimberlite) and are located off-craton (not truly adhering to Clifford’s Rule). The Canadian case illustrates exploration in heavily glaciated terrain. Here the diamonds were distributed far from their sources, and the most important discovery aspects were perseverance and scientific method.
In both cases, systematic exploration led to the discovery of many potential diamond host rocks (more than 80 kimberlite pipes in the Kimberley craton, and more than 300 in the Slave craton), only some of which were diamondiferous (10% and 50%, respectively). Even fewer were of sufficient diamond grade to mine (none in the Kimberley craton and less than 1% in the Slave craton). Taking into account how difficult it is to find just one kimberlite buried below surficial deposits and vegetation, these diminishing percentages illustrate just how special an economically viable deposit is. With the exception of the Canadian discoveries of the 1980s and 1990s (summarized in Kjarsgaard and Levinson, 2002), none of the producing countries have seen the discovery of a major diamond-bearing kimberlite pipe in recent decades. When this scarcity of discoveries is combined with the finite lifetime of existing mines, sometimes as brief as 25–30 years with modern mining techniques, a future shortage of rough diamond production could result.
ORIGIN OF DIAMONDS
Since the summary by Kirkley et al. (1991) that appeared in this journal, there have been major advances in understanding the relationship between diamond types and their hosts, the pressure and temperature conditions for diamond formation, the sources of carbon, and how diamond growth relates to fluids in the mantle. Much of this new information has centered around:
- (1) Better geologic dating on the mineral inclusions in diamonds in relation to the ages of depletion/metasomatic processes in the mantle
- (2) Refined models for the partitioning of major elements between the main minerals in mantle rocks
- (3) Improved analytical sensitivity, permitting spatially resolved, spot-sized chemical analyses for stable isotopes and trace elements
- (4) Realistic laboratory simulations of the behavior of carbon-bearing fluids in the mantle
- (5) High-resolution imaging and analysis of nanophases in microscopically transparent diamonds
Host Rocks for Diamond Crystallization and Diamond Types. Eclogite and peridotite are the chief rocks in which diamonds grow in the mantle (figure 18). Single diamonds in kimberlite are thought to be released from eclogite or peridotite by mechanical disaggregation during eruptive transport (Kirkley et al., 1991; Harlow and Davies, 2005). Whereas eclogites, diamondiferous or otherwise, survive transport by kimberlite, nearly all peridotite xenoliths are diamond-free. Diamond within a few eclogites has been studied by computerized axial tomography (CAT-scan) techniques. There diamond is found in between the major silicate minerals along pathways where metasomatic fluids usually traveled (Keller et al., 1999; Anand et al., 2004), although it is unknown whether diamond always has this spatial relationship. Diamond in peridotite may have a similar textural relationship with its major silicates, but because CO2-rich diamond-forming fluids react with magnesian silicates to form friable magnesite (MgCO3) along grain boundaries, diamondiferous peridotites disaggregate and release their diamonds, destroying the textural relationship with their host. Little is known about where, texturally, superdeep diamonds reside in their mantle hosts. At the high pressures and temperatures of the mantle transition zone and below, and in a mantle that is mobile by solid-state convection, they are not likely to reside in open cracks. They could, however, form in anomalously fluid-rich regions of the deep mantle.
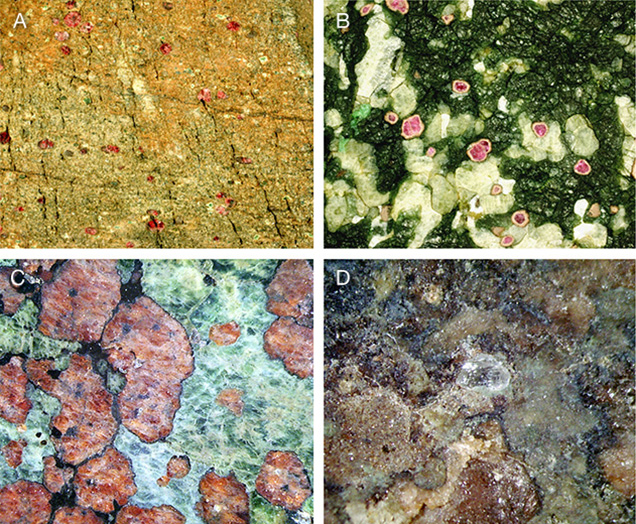
Diamonds that formed in the crust are restricted to terrains exposed by continental tectonic processes (again, see figure 4). They are found directly within their host lithologies, which are typically carbonate-bearing packages of rocks, including garnet-biotite gneisses and schists, which get reduced to form diamonds in place. Diamond also forms in the crust during the high pressures and temperatures produced when an extraterrestrial body strikes the earth’s surface to form impact diamonds from carbon-rich targets (graphite to diamond solid-state transition) or impact melts. Crustal diamonds are either small or not of gem-quality, and useful only to the abrasives industry.
The gemologist is taught the basic “type” classification of diamonds by nitrogen content and aggregation (Breeding and Shigley, 2009). This is very useful for gemology, but not for understanding diamond in its geologic context. Some 95% of natural lithospheric diamonds are type Ia. With the exception of sublithospheric diamonds, which are mostly type II, the remaining 5% non–type Ia specimens are poorly correlated with geologic setting. The composition of mineral inclusions in diamonds, even though more than 95% of monocrystalline diamonds are devoid of them, provides a more useful scheme because inclusion mineralogy can be closely related to the host rock.
Inclusions of silicate minerals (garnet and pyroxene) in lithospheric diamonds allow gem diamonds to be classified into two dominant groups following their major eclogitic and peridotitic host rocks in the mantle (figure 19). Silicate minerals transmit light and their compositional differences produce striking color variations, making these inclusions an effective way to classify diamonds into types. Compositional parameters akin to those used for indicator minerals in exploration are used to make the classification. Peridotitic diamonds are known as “P-type” and eclogitic diamonds as “E-type.” P-type specimens can be further subdivided into harzburgitic and lherzolitic in descending order of abundance that parallels the occurrence of these types of peridotites in the population of mantle xenoliths in diamondiferous kimberlites.
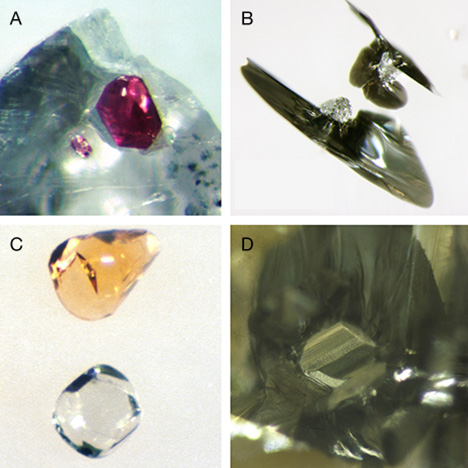
Sulfide mineral inclusions such as pyrrhotite and pentlandite (again, see figure 19) also allow diamonds to be subdivided into P- and E-types in a manner analogous to silicate inclusions. For sulfides, the distinction is based on Ni content. The higher-Ni sulfides occur in P-type diamonds, while the lower-Ni (and slightly higher-Cu) sulfides occur in E-type diamonds (Pearson and Shirey, 1999). Recent isotopic work using the platinum-group element osmium on single sulfides has yielded osmium abundance data that show the P- versus E-type distinction even more clearly (Pearson and Shirey, 1999). Unfortunately, the opacity of sulfides and their obscuration by internal fracturing renders this classification scheme useful only after breakage of the diamond and removal of the mineral inclusion.
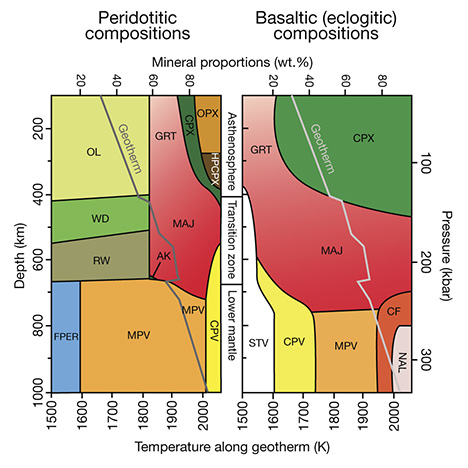
Mineral inclusions in sublithospheric diamonds are less understood than in lithospheric diamonds because of the rarity of specimens, the small grain size of inclusions, and difficulties in recognizing original high-pressure minerals from their low-pressure forms. But the basic distinction (figure 20) between peridotitic diamonds is basically related to Mg-rich, ultramafic mineral assemblages (such as Mg-perovskite, ringwoodite, wadsleyite, and olivine with ferro-periclase, majorite, and Ca-perovskite); in eclogitic diamonds it is related to basaltic mineral assemblages (such as majorite, clinopyroxene, CaTi-perovskite, Ca-perovskite, Ca-ferrite, stishovite, and the Na- and Al-bearing phase). These distinctions, which seem to apply to the deepest diamonds (Pearson et al., 2003; Shirey et al., 2013) will be fundamental in understanding the source of recycled materials in the deep earth.
Pressure-Temperature Conditions. All diamonds at the earth’s surface today exist outside the pressure and temperature regime for their growth. Yet we know the conditions under which they can form, based on experimental studies that simulate diamond-forming reactions, the mineral inclusion indicators of pressure and temperature (geothermobarometers), and the co-occurrence of diamonds in kimberlites with xenoliths whose pressure-temperature history can be studied. Basically, most gem diamonds are thought not to form directly from graphite. They can form at pressures and temperatures higher than the graphite-to-diamond phase transition under the right reducing conditions, and when there is enough free carbon to allow diamond to form. With depth below the surface, rocks reside at ever higher pressure and temperature along the geothermal gradient. This renders the entire mantle below about 140 km capable of forming diamond.
Pressure-temperature estimates for the formation of lithospheric, gem-quality diamonds can be calculated from analysis of their mineral inclusions. Review studies of more than 1,000 diamonds (e.g., Stachel and Harris, 2008) show that they formed at a temperature of 1150–1200°C and at a depth within the appropriate P–T range for diamond growth. Rather than reflecting a favored condition for formation, this may simply represent the most “probable” temperature range within the limits imposed by diamond stability and mantle conditions. Superdeep diamonds obviously form in a pressure-temperature regime much higher than what can be obtained in the lithospheric mantle, but one that can be estimated from the solid solution of silicon (the pyroxene or “majorite” component) into the garnet structure (Shirey et al., 2013), or some other estimate of minerals that have exsolved at low pressure from a higher pressure inclusion (e.g., Walter et al., 2011). Crustal diamonds have formation conditions best estimated from the metamorphic history of their enclosing host rocks, because they typically lack mineral inclusions that indicate pressure and temperature. Impact diamonds, forming directly at the earth’s surface, can only be modeled from the heat and pressure effects generated by the transient shock wave of the impact.
Sources of Carbon. Major advances in understanding the sources of carbon that eventually turn into diamond have come from examining carbon’s isotopic composition. Elemental carbon is composed of two stable isotopes: 12C (98.9%) and 13C (1.1%). The ratio of 13C to 12C, measured easily with a gas-source mass spectrometer, varies with geologic process and the original source of carbon. Nitrogen, the second most abundant element in diamond, also has two isotopes, 14N (99.6%) and 15N (0.4%), and is amenable to the same kind of study; however, because nitrogen is a trace element in diamond and much more difficult to analyze accurately, it has been subjected to far less scrutiny and less is known (for a recent review, see Cartigny and Marty, 2013). In general, isotopic study remains a very active area of research, because of the potential for diamonds to trace the igneous rock aspects of the deep mantle portion of the carbon cycle. Earth’s large solid volume relative to the atmosphere makes the mechanisms by which carbon contributes to the volcanic CO2 gas flux a critical factor that requires better understanding.
Carbon that becomes diamond has two sources in the earth: primordial and recycled. Primordial carbon is that which has resided in the mantle since the accretion of the planet. Recycled carbon has, at some stage, been released from the mantle to form CO2 in the atmosphere, or become incorporated in organic matter and formed carbonate, graphite, or other carbon-bearing minerals in sedimentary and metamorphic rocks. Carbon is a volatile element, and much of the original carbon available to be incorporated in the earth may have been lost during accretion (Marty et al., 2013). Thus, the amount of carbon in the mantle is not known (estimated at 500–1000 ppm; Marty et al., 2013), nor is the proportion of primordial to recycled carbon (given the large meteoritical range; Haggerty, 1999). We can say that carbon has a long residence time in the mantle (similar to the earth’s lifetime), that it has a concentration that may be close to steady state (i.e., unchanged by addition from subduction or loss due to volcanism), and that it is an actively cycled element. It is remarkable that diamonds display a large range in their 13C/12C isotope ratio despite the mantle mixing process of convection that might lead to isotope homogenization. In fact, diamonds retain compositional variability that is as large as the range induced by photosynthesis at the earth’s surface (the largest range in 13C/12C routinely measured).
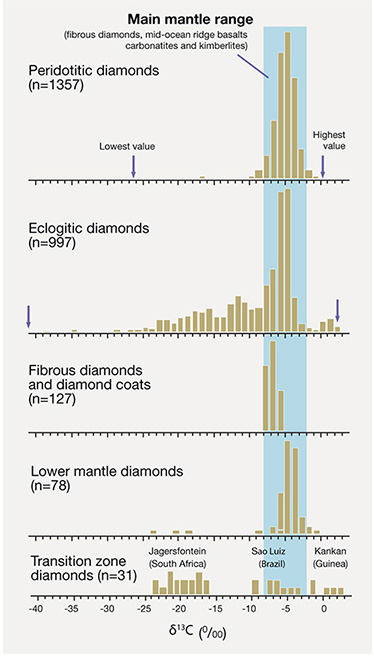
The variability in carbon isotopic composition is not random; instead, it is related to the diamond type and presumably to the petrogenesis or igneous geologic history of the diamond (figure 21). P-type diamonds display a restricted range in carbon isotopic composition (given in the delta notation, δ13C, where 13C/12C is referenced to a standard and expressed in ‰) of –10 to –2 δ13C, with more than 95% of P-type diamonds falling in the main mantle range of –8 to –2 (Cartigny, 2005). E-type diamonds show a very wide range in δ13C, from –42 to +3, even though they too have a large percentage that fall within the main mantle range.
Superdeep diamonds from the top of the lower mantle have a carbon isotopic distribution similar to that of P-type diamonds, whereas superdeep diamonds from the mantle transition zone more closely resemble E-type diamonds (Pearson et al., 2003). Other unusual specimens such as fibrous, polycrystalline, and crustal metamorphic diamonds have their own unique compositional ranges (Cartigny, 2005). Carbon in meteorites has a very large range in 13C to 12C that was inherited from solar system sources (Haggerty, 1999) and is much greater than that of the average mantle. If the carbon isotopic variability were inherited from primordial carbon with compositions even close to the large range seen in meteorites, then it remains unexplained how groups of diamonds would retain different compositional distributions and not all reflect that large range. A more likely scenario is that the compositional differences have been created by active geologic processes such as those related to plate tectonics.
Active geodynamic processes create and bring to the surface the diamonds we have today, just as they have shaped the earth for 4.5 billion years. These processes formed the ancient continents with mantle keels ready to store diamonds. They also introduced, deep in the earth’s interior, mobile substances such as water and carbon that were essential for the creation of diamonds and their sampling by kimberlitic volcanism. Oceanic lithospheric plates are eventually subducted, and when the oceanic lithosphere is created at the mid-ocean ridges by the decompression melting that occurs during spreading, hydrothermal circulation of seawater alters the primary minerals and deposits hydrous minerals in their place. Sedimentary carbonate minerals (high δ13C) and organic compounds (very low δ13C) collect in the oceanic lithosphere, along with volatile elements such as chlorine, fluorine, sulfur, and nitrogen. Since subduction must occur along the pressure-temperature path of the geothermal gradient, none of these volatile elements and compounds can be transported to the conditions of diamond growth as a fluid; otherwise, they would be driven off by the high temperatures reached. Rather, these elements must become locked, in solid form, in mineral structures (e.g., graphite, apatite, and biotite) or dissolved as trace elements within stable minerals (e.g., hollandite) that can be subducted to great depth.
One of the most active areas of current research is the distribution, speciation, and mineral hosts of volatile species in the oceanic lithosphere, and their transformations with pressure and temperature as the oceanic lithosphere is subducted. A goal for understanding diamond formation is to be able to predict the minerals involved and estimate their water- and carbon-carrying capacity. This is challenging enough for the earth’s current geodynamic regimes, where the present lithosphere can be sampled and geophysics can be used to form a picture of lithospheric plates and their rates and depths of subduction. But for understanding gem diamonds, most of which are billions of years old, an even greater challenge is whether current geodynamic processes are like those of the past. Despite these unknowns, the spatial association of old diamonds of different types with the rocks of known geologic history allows us to understand past processes at the basic level and to investigate the role of primordial versus recycled carbon.
Mantle convection, in which subduction plays a part, is the key process driving plate tectonics. Deep in the mantle, convection will trigger adiabatic or decompression melting, which occurs when the hotter mantle from below is convected upward too quickly to exchange heat with the surrounding mantle through which it moves (again, see figure 7). Due to the lower pressure of the shallower level it has reached, it is now hotter than its melting temperature at that pressure and will melt. Associated with mantle convection is the subduction of oceanic lithosphere. Subduction will enable the recycling of carbon as carbonate (CO3), leading to the creation of carbonated peridotite. Carbonated peridotite melts more easily than carbonate-free peridotite, and upon small degrees of melting will release a low-viscosity melt known as a carbonatite. Carbonatites are carbonate-rich igneous liquids that have too much oxygen to stably host a reduced carbon mineral such as diamond. They are, however, extremely mobile, and can move through parts of the mantle where they can be reduced and produce diamonds. Furthermore, carbonatitic liquids show compositional continuity with kimberlitic liquids (Gudfinnsson and Presnall, 2005), and it has been proposed that carbonatites will dissolve enough of the silicate mantle through which they pass to actually form a kimberlite (Russell et al., 2012). Thus, it is evident that the earth’s active geodynamics are intimately associated with all facets of the diamond cycle, including creation of carbon-rich regions where diamonds form, production of carbonated peridotite that can melt, the generation of the mantle upwelling that leads to melting, and formation of the kimberlite.
Fluids, Textures, and Diamond Growth in Mantle Rocks. The marriage of imaging techniques that can reveal diamond growth patterns with small-spot-size analytical capabilities has led to new ideas about how they grow in the mantle. Some work at the small scale borrows from the field of nanotechnology, and includes high-tech procedures at the cutting edge of resolution and sensitivity that involve laser ablation, secondary ion mass spectrometry, and focused ion beam fabrication and extraction of tiny diamond wafers.
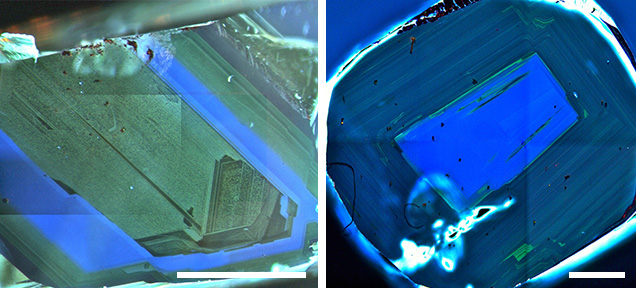
Such analytical work starts with the internal textures. Gem diamonds that show no growth zoning in visible light may show it in polarized light, photoluminescence, and especially cathodoluminescence (CL), as shown in figure 22. Nitrogen, the major diamond impurity, activates CL in diamond; thus, CL can be applied to almost every sample. It is best accomplished on polished plates, which must be oriented perpendicular to one of the {110} axes and not parallel to {100} or {111} to cut across the growth zones and display them (Bulanova et al., 2005).
Irregular forms of lithospheric diamonds (macles, bort, and the like) exist, but many monocrystalline lithospheric diamonds have a roughly concentric internal structure. In gem-quality monocrystalline diamonds, the zoning patterns are characterized by two chief features: (1) extremely thin oscillations between stronger and weaker luminescence, and (2) alternating episodes of resorption and overgrowth on top of the resorption. Both features strongly support the idea that diamond grows from an aqueous fluid and/or low-viscosity melt with an aqueous component rather than from a solid medium such as graphite. Growth from graphite is not likely under the P-T conditions of the lithospheric mantle for monocrystalline diamonds (Stachel et al., 2009), and it would not produce the fine oscillations (e.g., rapid change in nitrogen content) or periods of resorption between periods of growth. Zoning patterns are extremely important to interpreting carbon and nitrogen isotopic composition changes during growth and crystallization of individual mineral inclusions. Coated diamonds are a special case of monocrystalline diamond, where monocrystals have been overgrown by a thick, cloudy, polycrystalline coat laden with microinclusions of fluid. If the coat is composed of rods or blades of diamond, it will exhibit a fibrous structure and be termed a “fibrous” diamond. These coats are believed to grow during transport in the kimberlite and therefore represent young, new diamond growth surrounding often ancient diamond (Shirey et al., 2013).
The textures revealed in sublithospheric diamonds are strikingly different, because they rarely form euhedral monocrystals or (as seen with CL) display regular concentric zonation. Instead, these diamonds are characterized by multiple growth centers, non-concentric zonation of a blocky texture, and even what appears to be deformation texture; in short, they display almost polycrystalline internal structures. The major difference is that sublithospheric diamonds grow at much higher pressure and temperature and in a mantle that is actively convecting, whereas lithospheric diamonds grow in a mantle host that is not convecting. We can only speculate as to whether these textural differences are caused by the dramatic differences in the nature of the host mantle or by the possibility that some growth from solid graphite (Irifune et al., 2004) is favored by the much higher P-T conditions and deformation.
Diamond formation in the lithospheric mantle is considered a process whereby supercritical fluids or melts react with the mantle rocks through which they pass, a process known as metasomatism. A detailed discussion of this complicated topic is beyond the scope of this paper, but a brief review is necessary to understand general aspects of diamond growth. Diamond will crystallize when carbon is released from the fluid, either by the reduction of CO2 species or by the oxidation of methane (CH4) species. It becomes evident that the speciation of carbon and the formation of diamond will be intimately associated with the oxidation state of mantle rocks through which the fluids pass, which in turn is controlled by the mineralogy of the rocks and the type of reactions that ensue with the fluids/melts (Shirey et al., 2013). Thus, we can expect different diamond-forming reactions in peridotitic versus eclogitic host rocks. For example, a common reaction in peridotites involves enstatite and magnesite, which react to form olivine and diamond in the presence of a fluid. The mineralogy of an eclogite is different, so a comparable situation in an eclogite would involve dolomite and coesite to form diopside and diamond in the presence of a fluid. In both cases, CO2 is released by the system into the fluid, and diamond will only form if the oxidation state is low enough to allow it to be stable relative to CO2. The oxidation conditions for these different reactions in eclogite versus peridotite do not overlap, so that fluids too oxidized to form diamonds in peridotites are reduced enough to form diamonds in eclogite. Diamond-free fluids could pass through peridotite into eclogite to crystallize diamond. This process could explain the common occurrence of eclogite xenoliths with diamonds in metasomatic veins (e.g., Shirey et al., 2013).
At pressures where diamond is stable, cratonic lithosphere is likely to have sufficient reducing conditions for carbon to exist as diamond. A modern approach to diamond formation includes a comprehensive view of mantle oxidation state, carbon speciation in peridotitic and eclogitic mantle rocks, and both experimental and theoretical mechanisms for growth.
AGE OF DIAMONDS
Although some gemologists see beauty in mineral inclusions, a diamond with visible inclusions is of lesser value in the market. To the geologist or geochemist, a diamond with its mineral inclusion cargo is highly prized, because it preserves the oldest and deepest samples that can be obtained from the earth. Mineral inclusions are important for what they can tell us about the formation conditions (pressure + temperature), the host rock for growth, the source of diamond-forming fluids, and the age of a diamond.
Inclusion Types for Dating and Diamond Ages. Mineral inclusions can be classified as syngenetic, protogenetic, or epigenetic, according to when they crystallized with respect to their host diamond. Syngenetic inclusions crystallized simultaneously with the diamond, presumably in equilibrium with the diamond-forming fluid, and any geologic information extracted from the inclusion (e.g., P-T of formation, geochemical environment, and age) unequivocally applies to the host diamond. A protogenetic inclusion formed before the diamond and was encapsulated by it after some period that could vary from a geologically short to a very long time scale, and it could be related or unrelated to the diamond fluid. The maximum age obtained from a protogenetic inclusion might be close to the diamond’s age, and a general age pattern of diamond growth in a region of lithospheric mantle might still be evident. Inclusions forming along fractures or made of alteration minerals that formed after syngenetic or protogenetic inclusions can be identified as epigenetic. They are viewed as being secondary, and data from them on diamond crystallization is suspect.
P- and E-type inclusions of syngenetic and protogenetic nature from gem-quality lithospheric diamonds have provided most diamond ages to date (again, see figure 19). (The dating of sublithospheric diamonds is in its infancy, hampered because the inclusions are small and have unfavorable mineralogy for the commonly used radioactive decay schemes.) There is a large age difference between the relatively young kimberlites and the old lithospheric diamonds they transport. Diamondiferous kimberlites older than 550 Ma are rare, and most are younger than 150 Ma; the diamonds they carry are older than 1,000 Ma and may be as old as 3,500 Ma (again, see figure 10). With few exceptions, this age difference clearly negates a genetic link between kimberlite and diamond (see Kirkley et al., 1991), making the timing and origin of the host irrelevant to the timing and origin of its diamond cargo. The diamond cargo is just an accidental sampling of the ancient mantle lithospheric keel in which the diamonds were stored for long periods. The antiquity of lithospheric diamonds does make them ideal for probing the geologic processes occurring in the mantle keel during continent formation.
Inclusion Analysis Methods. Age dating of diamonds through analysis of their mineral inclusions has been reviewed regularly over the past two decades (Pearson and Shirey, 1999; Gurney et al., 2010; Shirey et al., 2013), and these reviews provide a source for the following discussion. Five isotopic decay schemes have been applied to the dating of these mineral inclusions (table 1): rubidium-strontium (Rb-Sr), samarium-neodymium (Sm-Nd), uranium-lead (U-Pb), argon-argon (Ar-Ar), and rhenium-osmium (Re-Os). All isotopic systems are classified as “long-lived” decay schemes, where the time it takes for the parent nuclide to decay to half its original amount is ideally suited to the old ages of diamonds.
Not all methods can be applied to every mineral inclusion; minerals have different abundances of the elements, and the analytical procedures themselves have different sensitivities. Breakthrough dating studies were made using the uranium-lead method on sulfide inclusions by Kramers (1979), and the samarium-neodymium method on silicate inclusions by Richardson et al. (1984), by combining all the available grains (sometimes many hundreds of diamonds, each with a single inclusion) of similar mineral composition (figure 23). This work established the validity of the procedures and generally demonstrated an Archean age (more than 2.5 billion years old) for the most depleted (harzburgitic) garnet inclusions in diamonds from the mines in the Kimberley area and Finsch in the Kaapvaal craton.
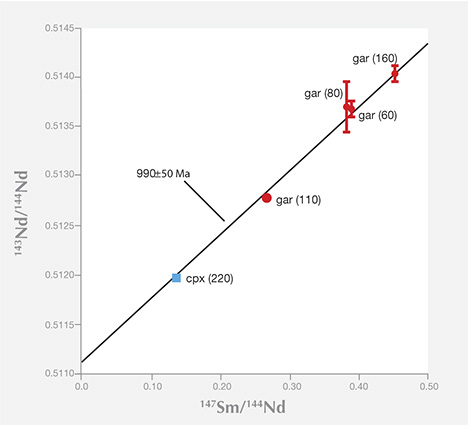
The use of batches of inclusions derived from separate diamonds led to concerns about mixing diamonds of different ages and producing an average age that might not correlate with a specific geologic process (Navon, 1999; Pearson and Shirey, 1999). These uncertainties drove the need to perform chemical analyses on single inclusions. The argon-argon method on clinopyroxene inclusions was the first to be applied (Phillips et al., 1989; Burgess et al., 1992) because eclogitic omphacite (a sodium-bearing clinopyroxene) contains sufficient potassium to allow age determinations. Although argon-argon geochronologic studies of eclogitic pyroxenes generally confirm the results of the samarium-neodymium method, problems arose due to diffusion of argon out of the inclusion to the surface where the inclusion and diamond meet. Nonetheless, argon-argon studies indicated not only E-type formation from the Neoarchean (about 2,800 Ma) onward in southern Africa but also some unexpected old diamonds, highlighting the need for work on single diamonds.
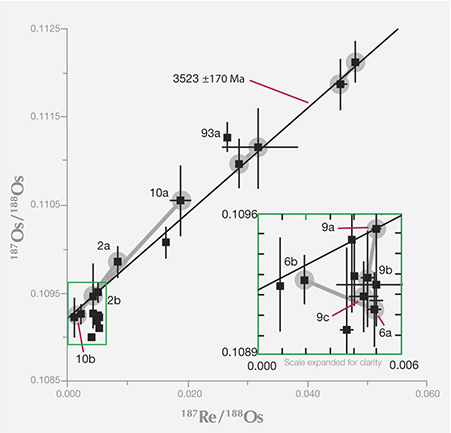
Analysis of single inclusions is now chiefly carried out using the rhenium-osmium isotope system in sulfides (table 1). The relatively high sensitivity of mass spectrometry techniques for Re and Os, and their relatively high concentration in sulfides, makes single-grain analysis possible. P-type sulfides weighing as little as a microgram (figure 24) can be analyzed due to their extraordinarily high osmium concentrations (Westerlund et al., 2006; Smit et al., 2010). But E-type sulfides have much lower concentrations (by as much as 1,000 times), and the focus on these mineral inclusions that are larger and easier to analyze has led to an apparent bias toward dating studies involving E-type diamonds (e.g., Pearson et al., 1998; Richardson et al., 2001; Richardson et al., 2004; Shirey et al., 2004a, b; Aulbach et al., 2009). Nonetheless, the single-inclusion work on sulfides by the rhenium-osmium method, with its ability to analyze a wide range of P-type and E-type sulfides, has led to important conclusions about the relationship of diamond growth episodes to processes that form and modify cratons (see below).
To date, only three age determinations have been made on inclusions from ultra-deep, sublithospheric diamonds, and all have come from the Brazilian craton. The uranium-lead method was used on one Ca-silicate perovskite inclusion (re-equilibrated to walstromite) from the Collier-4 kimberlite (Bulanova et al., 2010) and gave an age of 107 Ma, just slightly older than kimberlite eruption. A preliminary rhenium-osmium method was used on a sulfide inclusion from Juina and gave an early Proterozoic mantle model age around one billion years (Hutchison et al., 2012). Age inferences can be drawn from the Sr and Nd isotopic composition of majoritic garnets from Sao Luis that fall on the present oceanic mantle isotopic array (Harte and Richardson, 2011), which is known to often display Phanerozoic (e.g., 0–542 Ma) mixing ages. The point is that these ages are significantly younger than those of nearly all lithospheric diamonds.
Diamonds of Multiple Ages from Some Kimberlites. With the advent of widespread single-mineral inclusion analyses, it has become possible to see, with better resolution, if there is more than one episode of diamond formation at any one locality. Early work on E-type silicate inclusions clearly showed this possibility, but the need to combine many different grains from different diamonds always raised the possibility of combining formation ages as well. The general picture of lithospheric formation revealed by several generations of age dating, and the advent of the rhenium-osmium dating, is that there can be multiple diamond ages within the lithosphere sampled by any one kimberlite; perhaps this is the rule rather than the exception (Pearson et al., 1998; Richardson et al., 2004; Aulbach et al., 2009). For example, the Orapa and Jwaneng kimberlites carry three and four generations, respectively, of E-type sulfide-bearing diamonds, whereas the Diavik kimberlite carries Paleoarchean P-types and Paleoproterozoic E-types. The Ellendale kimberlite carries one P-type and three E-type generations. And of course, lithospheric diamonds are always found accompanying sublithospheric specimens because they are erupted in the same kimberlites, perhaps illustrating the greatest possible contrast in age and geologic setting.
Residence Time in the Mantle. Extensive samarium-neodymium studies by Richardson (summarized by Pearson and Shirey, 1999; Gurney et al., 2010) confirmed the general antiquity of lithospheric diamonds and their billion-year or longer residence in the lithospheric mantle, and established the Proterozoic as an important time of formation, at least for the Kaapvaal craton. Within the population of old lithospheric gem diamonds, some patterns emerge. The E-type sulfide-bearing diamonds analyzed thus far appear to have formed no earlier than three billion years ago (again, see figure 10). In contrast, diamonds containing P-type sulfides and silicates may be older or younger than three billion years. The geodynamic implications of these differences in residence time are discussed below.
This long lithospheric residence time for diamonds, as shown by all the mainstream studies with the samarium-neodymium and rhenium-osmium systems, contrasts sharply with a small number of studies in uranium-lead and argon-argon (e.g., Phillips et al., 1989; Burgess et al., 1992) on inclusions in lithospheric diamonds that have yielded relatively young formation ages due to analytical aspects of the particular system. Because the minerals analyzed can be related to proto-kimberlite melts, these ages are part of a growing body of evidence, supported by nitrogen-aggregation systematics, that a small proportion of lithospheric gem diamonds grew shortly before kimberlite eruption and occur mixed in with the much more abundant older diamonds in any one kimberlite.
The few sublithospheric specimens that have been analyzed are younger than most lithospheric diamonds. The uranium-lead on Ca-perovskite of 107 Ma was only slightly older than the age of kimberlite emplacement 93 million years ago, and was consistent with the highly aggregated nitrogen; this indicates a brief, hot residence time in the mantle (Bulanova et al., 2010). A model age of around 500 Ma, resolvably older than kimberlite ages but again much younger than lithospheric diamonds, was obtained with rhenium-osmium on a sulfide inclusion by Hutchison et al. (2012). This age is consistent with growth deep in the convecting oceanic mantle. Furthermore, neodymium and strontium isotopic analyses of a composite of majoritic garnets are also consistent with oceanic mantle compositions (Harte and Richardson, 2011), and support their derivation from the convecting mantle. The restricted age information on superdeep diamonds compared to lithospheric diamonds, and its potential for estimating deep mantle convection rates, means that this is an area of continuing research.
MANTLE GEOLOGY AND DIAMONDS
A sustained focus over the years on diamonds and how they form has allowed researchers to turn the tables and use diamonds as indicators of geologic processes in the mantle rocks that host them. This type of research is standard fare in the fields of igneous/metamorphic petrology and meteoritics, where each rock’s composition and the age relations of its constituent minerals may reveal an important story. Diamonds have always had unique potential because of their antiquity and depth of formation. But because they occurred as isolated xenocrysts in kimberlite, a detailed understanding of how they form was needed to realize their potential as a record of deep-mantle geologic processes.
Imitating Diamond Fluids at Depth. Perhaps one of the best ways that diamonds can be used to inform us about the deep earth is to simulate their growth in realistic physicochemical models of their mantle host rocks. This field of endeavor, known as experimental petrology, has a core research goal of duplicating diamond formation in the laboratory (see Shirey et al., 2013) at the pressure, temperature, behavior, and composition of the various components involved in the natural setting. This experimental approach is an essential aspect of understanding mantle geology through diamonds, because in many of the world’s diamond-forming regions the kimberlites have failed to expose samples of diamond host rocks.
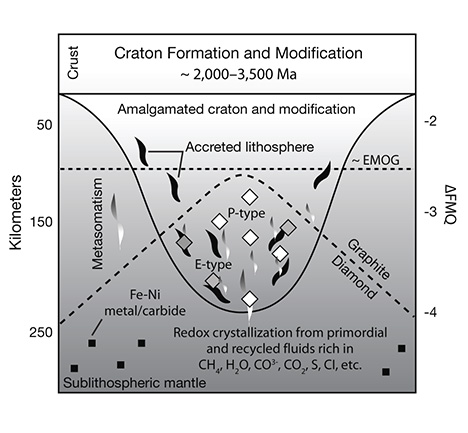
Diamond is the likely mineral form of free carbon (not bound up in other silicates) within the lithospheric mantle, leading to many ways that it can form, as briefly discussed above. From an experimental perspective, formation in the lithosphere will be in the compositional system carbon-oxygen or carbon-oxygen-hydrogen which will produce fluids that contain mostly CO2, mixed CO2 plus H2O, mostly H2O, and mostly CH4 (figure 25). Specific experiments and their results are too numerous to quote here, but various combinations of these fluids with carbonate or graphite in the presence or absence of silica or alkali metals have been shown to be effective in forming diamond. Sulfides and native metals also have been shown experimentally to foster diamond growth. Sulfides are common inclusions in diamonds, and metals are at least known. In the end, fluid/melt composition is likely to be important in facilitating or inhibiting diamond nucleation and perhaps in determining growth mechanism and crystal form. Although simplified systems are an insightful starting point, fluids and melts in the lithospheric mantle will react with silicate minerals in peridotite or eclogite, which can lead to a wide range of chemically diverse compositions as seen, for example, in fibrous diamonds.
In the convecting mantle below the lithosphere, diamond again will be the likely mineral form of free carbon. This region, which comprises the whole mantle above the core, has a silicate mineralogy that accommodates progressively higher pressure with depth. The olivine + orthopyroxene + clinopyroxene + garnet mineralogy of the lithospheric upper mantle gives way to a mineralogy dominated by wadsleyite + majoritic garnet in the transition zone and eventually aluminous silicate perovskite in the lower mantle (Harte, 2010; see figure 20). Because of the challenges of high-pressure experiments on these minerals, much of our current understanding comes from theoretical studies. The essential results of these numerous studies show that with increasing pressure, Fe2O3 (FeO1.5) is stabilized in the structure of these minerals over FeO. The net effect is to bind oxygen more greatly within the silicate bulk mineralogy of the mantle, reducing the rest of the minerals. Thus, the deep upper mantle and the entirety of the transition zone and lower mantle are expected to be reducing, metal-saturated, and potentially diamond-forming—even more so than the lithospheric mantle.
As in the lithospheric mantle, diamond again will form in the compositional system carbon-oxygen-hydrogen from fluids that are CH4- and H2O-dominated in the transition zone and shallow lower mantle and will become H2O-dominated in the deeper lower mantle (Frost and McCammon, 2008). At these incredibly high pressures, there is considerable storage capacity for hydrogen in the silicate minerals, which tends to suppress the existence of a free fluid and suggests that the carbon may be locked up in Fe(Ni) carbides (e.g., Fe3C and Fe7C3; figure 26). These phases may be stable enough to accommodate the entire carbon budget of the deep mantle for normal mantle regions that are not anomalously carbon-rich. Rohrbach and Schmidt (2011) recently proposed that the subduction of carbonate or carbonated peridotite to transition zone depths and below are an important way to add carbon to the deep mantle. If this were to occur often enough, carbonate reduction via the mechanism just outlined would be a ready way to make diamond.
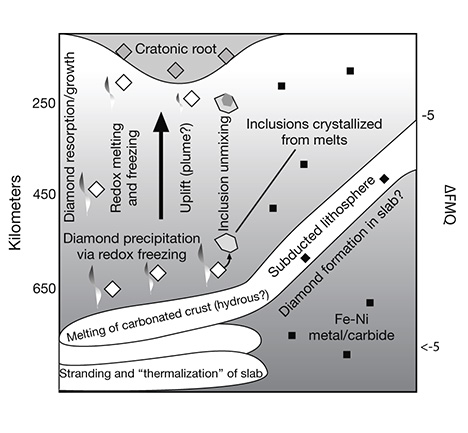
The thermodynamic and experimental observations described above permit a number of pathways for diamond crystallization, from a compositional range of fluids/melts. The compositional range of mineral and fluid inclusions found in diamonds likely attests to the importance of these pathways in nature, and perhaps involving both recycled and primordial carbon. What is clear from the range of mantle compositions is that diamond crystallization is an explicable and expected outcome of melt migration and mantle metasomatism. Future experiments will hopefully link sublithospheric inclusion mineralogy and trace-element composition to diamond fluid composition, deep mantle carbonate melt migration, and mantle reducing/oxidizing conditions in a way that can be related to mantle convection patterns (e.g., Walter et al., 2008).
Box A: The Kaapvaal and Kimberley Cratons |
The Kaapvaal craton in southern Africa was assembled about three billion years ago from two independent continental blocks by continental collision that thrust oceanic lithosphere under the western block. Fluids and sulfur carried under the western block by the hydrated oceanic lithosphere percolated upward, triggering a major pulse of diamond formation at the time of collision. The surface distribution of diamond ages and types is a direct result of this process. Mines in the western block all contain three-billion-year-old E-type diamonds, which are absent in the eastern block. Younger diamond formation in the Kaapvaal craton can be related to post-collisional events that modified the amalgamated cratonic block. In the center of the craton, igneous intrusion of the Bushveld Complex two billion years ago created the world’s largest storehouse of chromium and platinum-group metals. The parental mafic-ultramafic melts, originating below the lithosphere, passed through it before filling the Bushveld magma chamber in the upper crust. As the melts passed through the lithosphere, they left behind basaltic components. These basaltic components (eclogitic at these pressures in the lithospheric mantle) can be detected at present through their lowered seismic velocities in the region of the lithospheric mantle below the Bushveld Complex. This region of the lithosphere correlates with a greater proportion of E- versus P-type silicate inclusions, a greater incidence of younger (Mesoproterozoic era) Sm-Nd inclusion ages, a greater proportion of diamonds with light carbon isotope compositions, and a larger proportion of higher nitrogen-containing diamonds. The likely explanation is that diamond-forming fluids equilibrated with the preexisting silicate mineralogy of the lithospheric mantle and incorporated the silicates as inclusions, retaining the mineralogical differences imparted by the sublithospheric magmatism of the Bushveld Complex. The ability of fluids to form diamonds under wider reducing/oxidizing conditions in eclogitic rocks explains the correspondence of E-type diamonds with the seismically slow region, because some fluids unable to form diamonds as they pass through peridotite can still form diamond once they encounter eclogite. Subduction around the margin of the Kaapvaal craton was known to occur repeatedly (perhaps two to four times) throughout the Proterozoic era. Evidence for this is seen in the thermal and metamorphic history of rocks on the western and southern margins of the craton. The likely geometry of craton-margin subduction would have been to underthrust altered and thus fluid-rich oceanic lithosphere beneath the continental mantle lithosphere, allowing subduction-related fluids to invade the continental mantle lithosphere from below. Once these fluids encountered the reducing conditions of the continental mantle lithosphere, diamonds would form. Diamond mines in kimberlite that have penetrated such mantle lithosphere can sample multiple generations of diamonds. In the rather small Archean craton on northern Australia known as the Kimberley craton, diamonds formed differently. Here, continental lithosphere did not remain stable, yet it was a good host for diamond formation. The Kimberley craton is surrounded by zones of deformed crustal rocks known as mobile belts. The deformation evident in the crustal rocks must extend through to the continental lithospheric mantle because the continental crust will have mantle keel attached. Many of the lamproites and kimberlites that penetrate these mobile belts are diamondiferous, including two lamproites rich enough to be economic, Ellendale and Argyle. Here, the timing of diamond formation matches some of the deformation in the mobile belts. Sulfide inclusions in Ellendale samples carry three-billion-year-old rhenium-osmium isotope signatures attesting to the presence of ancient continental mantle keel in the mobile belt and its ability to host diamond growth despite evident tectonic activity. Other examples of intra-cratonic domains of younger Proterozoic mobile belt or magmatic arc terrain containing diamonds must exist; the Yavapai-Mazatlal terrane southeast of the Wyoming craton, and the Buffalo Head terrane southeast of the Slave craton in North America, may be examples of the same phenomena. The idea that diamonds can form beneath the younger mobile belts surrounding the ancient cratonic nuclei opens up new tectonic settings for exploration and ties some diamond formation to deep continent-scale geologic processes. |
Specific Mantle Geologic Settings. From the data gathered on diamond ages within some cratons, patterns of age and mineral inclusion composition can be linked to broad-scale regional cratonic lithosphere evolution. The way diamonds form in the lithosphere is better understood if diamond formation pulses can be correlated with thermo-tectonic events for which there is independent evidence. The best examples where this correlation can be drawn are the Kaapvaal craton of southern Africa (box A) and the Slave craton of Canada. In both cases, suites of diamonds that form with initial craton stabilization can be distinguished from diamonds produced by later fluids added to the base of the mantle keel by underthrusting of oceanic slabs or upwelling plume magmatism.
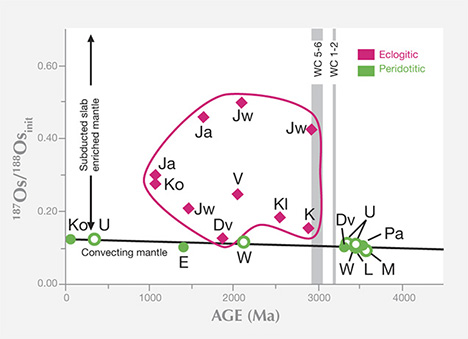
Correlation of Diamond Type with Geodynamic Processes. The diamond record remains one of the prime ways to examine geodynamic processes on the broad scale from mantle depths while avoiding the later overprinting effects of magmatism and metasomatism. A compilation of all the ages determined on lithospheric diamonds to date (figure 27) shows a significant difference in age distribution between E- and P-types. As a result of the association of the E-type paragenesis with eclogite, this difference can be interpreted to record the first capture at three billion years of high-pressure basaltic rock in the mantle keel of the continents. This process would have been intimately associated with ocean basin closure and continental collision (a process of modern plate tectonics known as the Wilson Cycle), because the basalt would have been derived from the ocean floor as it was underthrust, and incorporated into a portion of the mantle keel that thickened during collision. The absence of E-type diamonds before this time suggests that the process did not occur earlier, and may mark a transition from a planet dominated by more vertical geodynamic processes of plumes, recycling, and poorer crustal preservation to one dominated by lateral tectonics, subduction, and more efficient crustal preservation. Independent evidence from geologic studies of exposed crustal rocks supports such a dramatic change.
This proposed change in geodynamics may have important implications for the nature of carbon-bearing fluids and their delivery to diamond-forming depths in the mantle. Using subtle trends in the carbon isotopic composition of P-type diamonds, Stachel and Harris (2009) proposed that older specimens formed by methane oxidation and younger ones by carbonate reduction. If this observation is combined with the proposed onset of the Wilson Cycle, it could signify a change from the geodynamic processes that favor primary mantle devolatilization and/or the outgassing of recycled reduced fluids, to the geodynamic processes that favor carbonate recycling via slab subduction (Shirey et al., 2013).
Sublithospheric diamonds may be young enough to provide a unique way to follow the deepest parts of the mantle convection that drives current plate tectonics. Seismic studies, using a technique called mantle tomography, are able to image the subduction of oceanic lithospheric plates into the mantle transition zone (400–660 km depth), and in a few cases into the very top of the lower mantle (700–800 km). Mineral inclusions in sublithospheric diamonds from these depths can be grouped into those that have peridotite-like compositions, those that have basalt-like compositions (again, see figure 20), and those that are calcium-rich (Harte and Richardson, 2011). The first two groups of inclusions are suggested to form in diamonds whose source fluids/melts may have been generated by dewatering the subducting oceanic lithospheric plate, whereas the third group is thought to be produced in association with carbonatitic melts generated from carbonated peridotite. In many cases, the basalt-like inclusions have compositions indicative of a surface origin and are housed in diamonds composed of a significant amount of recycled carbon. The return of these minerals in kimberlite-erupted diamonds confirms the seismological evidence of subduction-recycling to the depth of the shallowest lower mantle (figure 26). Furthermore, association of some inclusions with carbonatite may suggest a connection between diamond formation in the deep mantle and the onset of plume initiation.
SUMMARY OF THE GEOLOGY OF DIAMONDS
The past 25 years have seen scientific research answer many of the basic questions about gem-quality diamonds. For example, we now know that diamonds are old—in many cases nearly as old as the continental mantle keel in which they are stored. We can relate their age to the age of their hosts—in some cases distinguishing different generations of diamond-forming events.
We also know that diamonds form from fluids melts whose composition and carbon speciation is controlled by the reduction-oxidation state of the rock through which they pass. We know that recycling of carbon may be essential, with the possibility that the recycled material may have changed through geologic time. Furthermore, the analytical tools exist to extend this knowledge and use diamonds as more sophisticated probes and tracers of deep mantle processes.
New Perspectives on Diamond Geology. Just as an enormous leap was made in the 1880s with the recognition that diamonds are found in kimberlite, recent advances have been made by putting together high-precision, high-resolution microanalyses of diamonds and their mineral inclusions, radiogenic and stable isotopes, geophysics, and the discovery of diamonds in unexpected new places. Clifford’s Rule, the prospector’s guideline that confines diamondiferous kimberlites to the Archean or oldest parts of the stable cratonic blocks of continental crust, worked well because of fortuitous geologic features. It is not only that the Archean was a special time to form diamonds—we have large numbers of samples formed in the Proterozoic. Rather, it is that in South Africa, the site of early geologically driven diamond prospecting, the craton was formed by an Archean continental collision that produced many diamonds at that time (figure 28). The collision made the mantle keel under the Archean crust deeper and more melt-depleted than the mantle keel under Proterozoic crust. Thus, it was just deep enough and reduced enough to receive much younger diamond-forming fluids created by subduction-related orogenic processes.
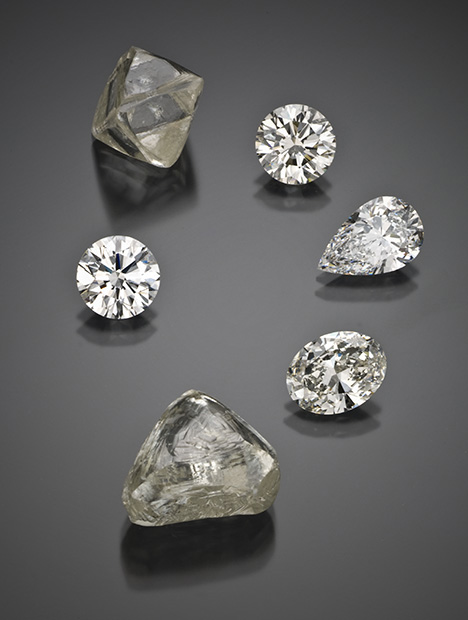
A new revelation, the result of dating both the diamonds and the depletion of their host mantle, is that diamond formation can appear associated with mobile belts, and that those intracratonic regions near Archean cratons may contain Archean mantle keel that was later remobilized. These could be ancient cratonic specimens that survived later tectonism in the mantle keel, or simply appear to be in a mobile belt because the latter was thrust onto the craton. A third possibility is that they could actually be younger diamonds formed from much older components. Indeed, the Argyle mine lies in just such a mobile belt, as do other very productive mines (e.g., Venetia) or diamond-rich localities (e.g., Sloan, Buffalo Head). Far from the simple correlation with just ancient crust, predicting where to look for diamonds must now include new thinking about the geologic evolution of the mantle lithosphere and the geologic sources of the diamond-forming fluids.
Ubiquitous Diamonds. Despite the rarity of gem-quality lithospheric diamonds in kimberlite and the rarity of kimberlite as a volcanic eruption, diamonds may not be as rare a mineral in deep sublithospheric mantle. The recent improvements in understanding the reduction-oxidation conditions of the deep mantle show that most of the mantle presents the right conditions for diamond to crystallize. In other words, diamonds should be ubiquitous. What typically keeps them from being more abundant is the lack of a geologic mechanism to put enough carbon in a free fluid/melt phase from which the diamond can crystallize. Where this can occur, diamonds will form. Another important aspect is that only a kimberlite (or lamproite) can bring them to the surface, so they may not be easily sampled from such depths. Diamonds may not be capable of surviving slow-ascending mantle plumes, and they certainly will be dissolved in the oxidizing magmas of basalt, alkali basalt, nepehelinite, and carbonatite that might be derived from them.
Tracing the Carbon Cycle. Diamond is recognized as the only material sampling the very deep mantle to depths exceeding 800 km, as shown in figures 25 and 26. Diamond is less useful at revealing deep carbon flux (the amount of carbon in motion) because it can provide only a small, variably distributed sample that is usually not directly related to its kimberlite host. Since we recognize diamond as deriving from a mobile carbon-bearing fluid/melt, it takes on new importance in tracking carbon mobility in the deep mantle via these fluids/melts. At the same time, diamond can be used to reveal mantle mineralogy and mantle reduction-oxidation state—actually exposing and preserving very tiny mantle minerals from these great depths. Through these mineral inclusions and the composition of the diamond, we have the unique ability to follow the path and history of the carbon from which it formed. Thus, diamond truly occupies a unique position in any discussion of the igneous and metamorphic aspects of the earth’s carbon cycle.
FUTURE RESEARCh
The advances of the last two decades have led to new conclusions about how diamonds crystallize and are stored in the mantle. We now know that they form from C-O-H-S fluids that flow through deep mantle rocks, especially in the lithosphere. These fluids transform the rocks by metasomatism while precipitating diamond. The reduction-oxidation state of the host rocks controls the diamond-forming reactions and can tie, locally and globally, geologic processes and mineral inclusion compositions to specific diamonds. Diamond has an internal growth morphology that records the chemical effects of this process and incorporates minerals during growth; this morphology can be used to study the deep mantle. Throughout the earth’s history, diamonds formed by a multiplicity of reactions, rather than just one. Diamonds are potentially widespread in the mantle, as opposed to their scarcity in kimberlites and indeed the scarcity of kimberlites themselves. Formation could have taken place in recent geologic times, and may even be occurring now. The study of diamond provides a way to study deep mantle convection related to plate tectonics.
As scientists, we want to know the source of the carbon from which diamond is composed. Is it primordial or recycled? If the carbon is recycled, how does it get into the pressure and temperature regime of diamond growth from a low-T, perhaps even mobile phase? If the carbon is primordial, what does its presence and distribution tell us about how it was accreted into the earth and stored since the earliest times?
Nitrogen is the most abundant element in diamond after carbon. What is its chemical nature, and how is it partitioned between the diamond and its fluid during growth? When a diamond grows, is the isotopic composition of the carbon and nitrogen maintained or changed? Herein lies the key to using the isotopic composition of these two elements as important tracers of a diamond’s geologic history.
Diamonds have long been grown by industrial processes, but we are just now capable of conducting geologically realistic growth experiments. How will the conditions of diamond growth relate to external morphological features or the incorporation of mineral components on the atomic scale, or the molecular makeup of the components in diamond-forming fluids?
In the deepest mantle, it is just now being understood that diamond formation may be related to highly mobile carbonatitic (CO3-dominant) magmas and regions where metal formation can remove the oxygen to leave reduced carbon ready for diamond crystallization. Could it be that diamond is an essential mineral link, not just an occasional participant in this aspect of the carbon cycle?
Diamond has a unique position as one of the earth’s oldest preserved minerals. How have all these processes, especially the nature of diamond-forming reactions, changed with time? The answers to these questions await the discoveries of the next decade.
GLOSSARY
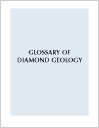
Download: Recent Advances in Understanding the Geology of Diamonds - Glossary (PDF)