Diamonds Are Not Forever! Diamond Dissolution
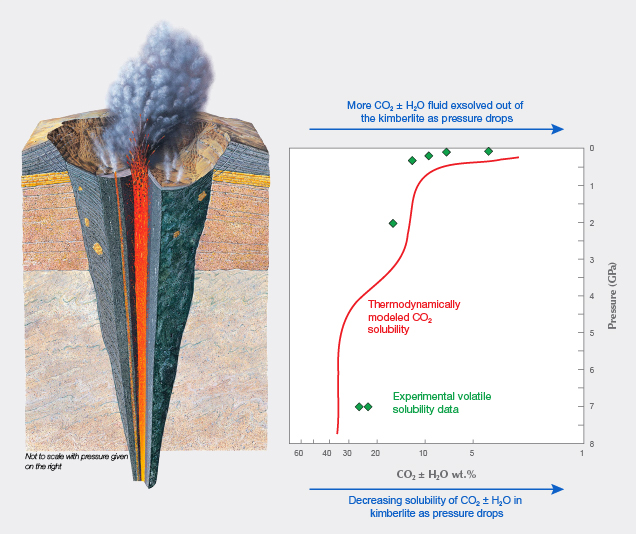
Before cutting and polishing, diamonds have highly variable surface features rarely, if ever, seen by the jewelry wearer. These features can tell an interesting story of diamond’s geological history deep within Earth—both in the mantle rocks where diamonds grew and during their subsequent volcanic transport. Our previous column showed that volcanic eruptions of kimberlite are how diamonds make their way from depth in the mantle to Earth’s surface. But this violent process does not leave the rough diamond unscathed.
These early histories are rarely considered once the diamond has been faceted and set into jewelry, but they raise interesting and geologically important questions:
- Why do rough diamonds look so different from each other, and what might this tell us about their geological history?
- What effect does the kimberlite magma have on the diamond cargo?
- How can we see through this later stage of the diamond’s history to its millions and billions of years of mantle storage?
Features on Natural Rough Diamonds
There is so much variety in natural diamond surfaces that, like snowflakes, no two diamonds are exactly alike. These differences can give us useful information about how diamonds react with fluids in the mantle after crystallization and also reveal the dissolving properties of the kimberlite magma that brought them to Earth’s surface.
External surface and internal features are related to the internal crystallographic structure of the diamond. Deformation lamellae (also known as “graining” to gemologists) can be subtle and evenly distributed. Other features relating to diamond structure, such as trigons, are only skin deep, microscopic, and can seem randomly distributed. The external form of a diamond crystal can be dissolved to form secondary shapes by the partial removal of crystalline diamond in a geological process known as dissolution or resorption. Left alone without dissolution, diamond will form a perfect octahedron or a cube. But with dissolution, diamond can change from an octahedron to other forms such as dodecahedron or tetrahexahedron, and even form “irregular” diamonds with no discernible shape.
These transformations to the rough diamond’s exterior surface can be distinguished from one another. Complex shapes can form in the mantle prior to being picked up by the kimberlite. Resorption to secondary shapes and trigon formation can occur during kimberlite eruption. Surface features like hillocks and frosting are also regarded as features imposed by the kimberlite. Finally, if released from easily weathered kimberlite, diamond lying in streambed deposits known as “placers” and in crudely sorted sedimentary rocks known as “conglomerates” can take on new surface features. Crystal rounding and percussion marks form when a diamond is transported in high-energy fluvial environments. Green and brown radiation stains are surface features that are due to a diamond’s proximity to radioactive fluids or minerals.
Dissolution During Kimberlite Transport and Mantle Storage
Unless a diamond is weathered out of kimberlite, kimberlite eruption will produce the last visible resorption effects, as it is the last event affecting the diamond before it reaches Earth’s surface. Features produced by the kimberlite will overprint or even remove those features produced in the mantle. Once kimberlite effects are studied and understood, we can peer beyond them to a diamond’s earlier history in the mantle. But why does kimberlite magma attack diamonds in the first place?
Successful diamond transport and delivery occurs because kimberlites erupt very fast (transiting 150–200 km in <10 hours to ~2 days; Russell et al., 2019). This rapid eruption means that any entrained mantle components—such as xenoliths and diamonds—lose pressure very quickly, are violently tumbled with upward movement, and can break apart.
Because kimberlites are more oxidizing than diamonds, they typically are in the process of dissolving diamonds—it’s just that this process has often not gone to completion. The chemical composition of the kimberlite and its volatile components (such as carbon dioxide and water) are important factors. For example, as pressure drops during ascent, the kimberlite magma is not able to dissolve as much carbon dioxide, and a fluid rich in carbon dioxide and water exsolves from the magma. (figure 1; Brey and Ryabchikov, 1994). This fluid, which often dissolves diamond, normally starts to exsolve at around 3–4 GPa (a gigapascal is a unit of pressure equal to 10,000 times atmospheric pressure), with maximum exsolution once the kimberlite is at pressures below 1 GPa.
The starting composition of the kimberlite melt at depth in the mantle affects the amount of carbon dioxide that can be dissolved in it, and hence the amount of carbon dioxide that will exsolve as it reaches the near-surface. Carbon dioxide solubility is significantly lower in water- and silica-rich kimberlites, meaning they will exsolve more carbon dioxide than water- and silica-poor kimberlites (Brooker et al., 2011; Russell et al., 2012; Moussallam et al., 2016). The amount of carbon dioxide that exsolves from the kimberlite melt at the near-surface will have a direct effect on the amount and style of resorption that the diamond cargo experiences.
Storage in the mantle is static for the most part and does not involve such drastic pressure changes. But we know that dissolution in the mantle occurs regularly since the internal structures of most diamonds show evidence for multiple growth episodes often intersected by periods of resorption (figure 2). We know from diamond ages, and the much younger kimberlite eruption ages, that diamonds reside in the mantle for millions to billions of years. Geochemical analysis shows that during this time, fluids pass through the mantle during a process known as mantle metasomatism. Mantle metasomatism seems to be similar to the process by which infiltrating fluids lead to diamond crystallization in the first place. Thus these fluids have the potential to react with the diamonds that are already there. Over this long duration in the mantle, there is the potential for many different carbon-bearing fluids/melts to contribute to diamond growth.
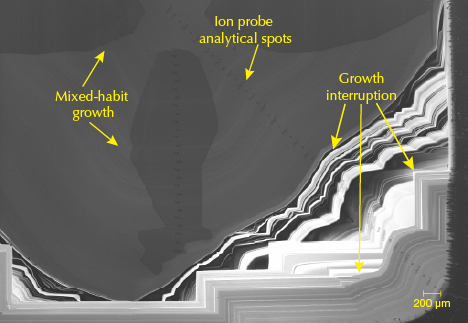
Timescales for Mantle Resorption/Dissolution from Radiometric Dating. In rare instances, diamonds with multiple inclusions concurrently crystallizing with different growth zones have revealed the timescales over which some diamonds grow in the mantle. A diamond from the Mir mine in Russia contains multiple sulfide inclusions in core and rim growth zones. The inclusions in each zone are hosted by diamond with vastly different carbon isotope compositions and have a core that differs by about 1 billion years from the rim, which is much greater than the age uncertainty (Wiggers de Vries et al., 2013; Bulanova et al., 2014). An even larger age difference of around 2 billion years was recorded by garnet and clinopyroxene inclusions in a diamond from the Letlhakane mine in Botswana (Timmerman et al., 2017). These growth events are intersected by periods of diamond dissolution/resorption, or otherwise by periods of stasis where neither growth nor resorption is occurring.
It is unclear whether such long periods between growth and resorption are normal or just rare examples. For example, the oldest diamonds ever, dated from the Ekati mine in Canada (Westerlund et al., 2006) with ages of 3.5 billion years, show older cores and younger rims that are likely separated by less than 100 million years of age. These zones may even have grown with a very slight age separation, perhaps almost contemporaneously.
Even the best age dating techniques we can apply simply do not have the precision to distinguish the small differences (e.g., <100 million years) in age when diamonds are often billions of years old. However, microscopic imaging techniques such as cathodoluminescence and DiamondView can reveal the growth layering that occurs internally in most gem diamonds. Based just on cross-cutting relationships and the superposition of younger diamond layers on top of older, preexisting diamond, we are sure that different generations of diamond-forming fluids exist. We can see what layer must have come first and what layer second, and that dissolution of preexisting diamond is part of the story.
Experiments Reveal Which Features Originate During Storage Versus Transport. The only way to find out whether features originate during mantle storage or kimberlite transport is to classify the type of dissolution features seen, and then to reproduce them experimentally to match the conditions in each setting. Dissolution experiments on diamonds have been conducted for decades, but many recent advances in our understanding of how different fluid compositions affect the resorption and features on diamond surfaces have been made by Yana Fedortchouk and Zhihai Zhang, working at Dalhousie University in Canada. For a detailed overview of the experimental literature on diamond dissolution, the reader is referred to Fedortchouk (2019) and Fedortchouk et al. (2019).
Their work has produced a roadmap of the dissolution features (figure 3), allowing gemologists and research scientists to peer into the complicated history of diamond revealed by its surface features. The scientific goal of such work is to understand the fluids that exist in the mantle and during kimberlite transport: If these fluids can dissolve diamond, they are also the kind of fluids from which diamond can grow.
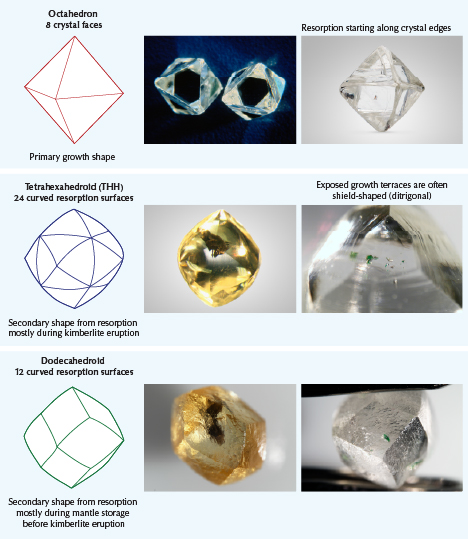
Dodecahedra are actually (at least) two different secondary shapes, and they should have names ending in -oid to reflect the fact that they have curved resorption surfaces that approximate similar crystallographic directions to true crystal faces.
Dodecahedroids have 12 curved resorption surfaces that approximate true dodecahedra. Tetrahexahedroids (THHs) have 24 curved resorption surfaces that approximate the shape of tetrahexahedra.
Dissolution experiments show that three main factors may impact the shape of a resorbed diamond (Fedortchouk, 2019; Fedortchouk et al., 2019). Different features can be produced by varying the depth of the fluid exsolution from the kimberlite, or through varying the Si content and temperature of melts in the mantle:
1. Whether a fluid phase is present or not. A fluid-free kimberlite melt will not lead to rounded resorbed THH. Instead, there is some resorption along the octahedral crystal edges and the development of “sharp” corrosive surface features (Fedortchouk and Zhang, 2011).
2. Depth of fluid exsolution from the kimberlite. A water-rich fluid at 3 GPa will favor rapid formation of THH, whereas the same fluid at 1 GPa will favor octahedra preservation (Zhang et al., 2015).
3. Amount of CO2 and H2O in the fluid. Experimental work by Fedortchouk et al. (2007) shows that at 1 GPa, there is higher preservation of the octahedral faces in a CO2-rich fluid. A water-rich fluid would favor formation of THH and elimination of octahedral faces. Since kimberlite magmas change composition during ascent, so does the magma’s effect on diamond resorption. Different resorption styles can be expected, even during the same eruption!
Tetrahexahedroid (THH). Experiments indicate that tetrahexahedroids (THH) form in water-rich carbonate melts (Chepurov et al., 1985; Khokhyrakov and Pal’yanov, 2007). Tetrahexahedroid crystals have been experimentally produced at 1.0–1.5 GPa by dissolution in natural kimberlite with 6.2 wt.% H2O and 4.8 wt.% CO2 (Kozai and Arima, 2005) and in experiments with H2O and CO2 fluids (Fedortchouk et al., 2007). Because they form during kimberlite eruption, THH diamond crystals are much more common than dodecahedroids, and their formation may overprint any mantle resorption features or shapes.
Dodecahedroid. Dodecahedroids form in CO2-rich carbonate melts that are poor in water (Khokhyrakov and Pal’yanov, 2007, 2010 and many references therein). They are thought to form mostly during dissolution processes in the mantle (Fedortchouk and Zhang, 2011). Dodecahedroidal shapes are more rare, as they can be overprinted to THH during kimberlite eruption. These shapes have a higher likelihood of survival if the kimberlite magma and its exsolving fluids are water poor, and also if diamonds are shielded from the kimberlite magma by enclosure in their mantle host rocks such as peridotite and eclogite.
Trigons
Examples of one of the most common surface details of rough natural diamonds—trigons—are shown in figure 6. Additional excellent examples can be found in the book Diamonds in Nature: A Guide to Rough Diamonds (Tappert and Tappert, 2011).
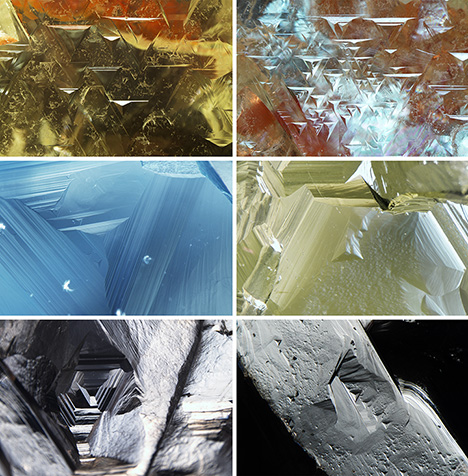
Trigons can be either “negative” or “positive,” where a “negative trigon” has an opposite orientation to the octahedral face of the diamond (figure 7). In the past, there was significant debate about whether trigons were growth features (e.g., Tolansky, 1955, 1960) or etch pits (e.g., Frank and Puttick, 1958; Patel et al., 1964; Lang, 1964). These days it is accepted that a trigon is an etch feature, produced when oxidizing fluids/melts start to dissolve the diamond. Dissolution typically starts at an imperfection, such as a lattice dislocation (Wilks and Wilks, 1991).
When Do Rare Positive Trigons Form? Positive trigons are extremely rarely seen. Snap Lake in Canada is one locality that has a high proportion of diamonds with positive trigons, and their development was attributed to near-surface reaction of the diamonds with late-stage, low-temperature C-O-H fluids (Li et al., 2018). This is consistent with early experimental work that showed that positive trigons are the most common etch feature at atmospheric pressure (Evans and Sauter, 1961). These early experiments also showed that the orientation of a trigon (positive versus negative) could be changed by using different etchants at different temperatures (Frank and Puttick, 1958; Evans and Sauter, 1961).
More recent experiments have shown that positive trigons can be produced by dry carbonate melts, and that increasing water content changes trigon orientation to negative (Khokhyrakov and Pal’yanov, 2000, 2010). Fedortchouk (2019) attributes positive trigons to resorption by a rare type of mantle fluid (rare since positive trigons are associated with other rare features such as hexagons and transverse hillocks). It is possible that the development of positive trigons is common in the mantle, and that they have just been “erased” by later resorption of the diamond into THH during kimberlite transport.
Influences on the Shape of a Trigon. Experimental work has shown that the shape of trigons is influenced by the amount of H2O and CO2 in the fluid, as well as temperature. Trigons produced by water-rich fluids have a limited size range but a large depth variation; in contrast, trigons produced by CO2-rich fluids can have varying sizes but always with a positive correlation between depth and diameter (Fedortchouk, 2015). Large trigons are produced at higher temperatures (Kanda et al., 1977; Fedortchouk, 2015). Additionally, experiments at 1 to 3 GPa showed that CO2-rich fluids mostly produced trigons with pointed and curved bottoms, whereas CO2-poor fluids produced mostly flat-bottomed trigons (Zhang, 2016).
The Importance of Understanding Resorption, and Remaining Questions
Since the surface features of diamonds are heavily influenced by the composition and temperature of the dissolving fluid/melt, these features are another tool that mantle geochemists can use to study carbon-bearing fluids/melts in the deep Earth. Actual fluids are preserved in fibrous diamonds, but these may be a specific subtype of mantle fluids and their universal applicability to all monocrystalline gem diamonds needs to be better established. Since very few diamonds actually contain fluids, the study of fluids resulting in surface features on a wide variety of different diamonds may provide a broader understanding of carbon fluids in the deep Earth. Furthermore, there is a need to understand diamond resorption styles during the economic evaluation of kimberlite since complete resorption can destroy diamond grade by eliminating diamonds altogether. Identification of diamond quality in each kimberlite unit leads to better decisions about which kimberlite units to prioritize during mining.
When does a fluid result in diamond growth, and when does it dissolve diamonds? We need a better understanding of how diamonds are resorbed and destroyed in the mantle. Carbon-bearing fluids in the mantle seem to be largely responsible for diamond growth (e.g., Stachel et al., 2017) and do not contribute to diamond dissolution at high pressures during mantle storage (Fedortchouk et al., 2019). Conversely, carbon-bearing fluids exsolved at low pressures (figure 1; less than 3 GPa) during kimberlite eruption do dissolve diamonds to form rounded THH (figure 5; Fedortchouk et al., 2019). In the mantle, the main diamond dissolution agents seem to be carbonate melts that can produce several features through variation of silica contents and temperature (Fedortchouk and Zhang, 2012; Fedortchouk et al., 2019).
We have shown that diamond can be dissolved during two periods of residence within the earth: where it grew in the mantle and during its volcanic transport to the surface. Given that diamond dissolution is common, it is fortuitous that the mantle’s temperature, high pressure, and low oxygen state makes it friendly for diamond growth. Similarly, given that diamond dissolution can occur in fluid-rich kimberlites, it is a good thing that transport is fast enough to overcome the speed of dissolution. In the end, given that diamond dissolution is common in two settings in Earth, it is rather miraculous that diamonds do actually survive. Here we have yet another way in which natural diamonds are remarkable.