Laboratory Growth of Gem Materials and the Attempt to Replicate Nature
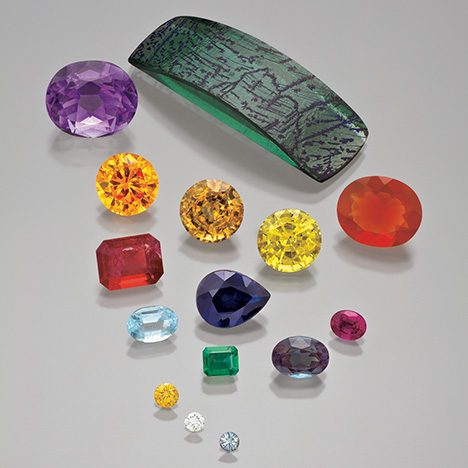
Our last three installments of Colored Stones Unearthed have focused on geological processes that form gemstones naturally in the earth. We detailed gemstones formed in magmatic, metamorphic, and sedimentary geological environments. The separation of geologic processes into those involving molten rocks (magmatic), solid-state recrystallization at high pressure and temperature (metamorphic), and deposition and lithification of sediments (sedimentary) might seem intuitive to any modern student of geology. For most of human history, however, the forces involved in shaping the earth and forming these precious gemstones were almost entirely unknown.
Although laboratory-grown (synthetic) gemstones are commonplace today, the technological breakthroughs that enabled them were not feasible until scientists unraveled the geologic mysteries behind gem formation. This knowledge allowed researchers to replicate these conditions in a laboratory setting (figure 1). Early attempts to artificially reproduce the beauty of natural gemstones were mostly relegated to glass imitations or primitive treatments applied to natural stones. Laboratory processes for growing gemstones are very sophisticated today, but it was not until the 1800s that humankind was able to imitate, in some way, the geological processes that formed precious stones naturally. And yet, for the most part, the technology has not advanced far enough to perfectly replicate the gemological properties of natural stones using artificial growth methods. As a result, most synthetic colored stones are easily detectable by a gemological laboratory.
This installment of Colored Stones Unearthed gives an overview of processes for growing gem materials in a laboratory and how differences in the synthetic and natural growth environments enable laboratory-grown stones to be separated from natural materials. Note that this is not intended as an exhaustive overview of criteria to separate natural and laboratory-grown stones, nor is it an exhaustive overview of current methods for laboratory growth. Rather, it is meant to serve as a comparison between natural and artificial processes for the formation of gemstones. For historical reviews of crystal growth methods, see Bohm (1985) and Feigelson (2015, 2022).
GEOLOGICAL PROCESSES FORMING GEMSTONES
The geological processes forming natural gemstones can be roughly divided into magmatic (Palke and Shigley, 2022), metamorphic (Palke and Shigley, 2023), and sedimentary (Shigley et al., 2023). Magmatic gemstones are those that formed through igneous processes involving molten rocks, or magma, somewhere within the earth, likely in the crust or upper mantle. Examples include some sapphire and peridot associated with alkali basalts, some feldspar, some garnet, and topaz when including crystallization from vapor phases in cooling rhyolitic lava flows. Conditions may vary, especially the depth of formation, but the temperatures involved are generally at least 750°C, which is roughly the minimum temperature at which rocks composed of silicate minerals will begin to melt and form magma that can separate to form a free magma phase.
Metamorphic rocks, in the strictest sense, form by heating and compression of rocks in the solid state, recrystallizing the rock and forming a new texture and possibly new mineral phases. In reality, metamorphic processes rarely happen entirely in the solid state but involve some type of fluid flow, facilitating the transport of chemical components. Metamorphic gemstones include many rubies and sapphires, jade, tsavorite, tanzanite, and various garnets. Pressure and temperature conditions for metamorphic ruby and sapphire typically range from 2 kbar and 550°C to about 11 kbar and 825°C (figure 2).
Sedimentary gemstones are classified as those that formed not necessarily directly through sedimentation and compaction but rather through low-grade diagenetic processes, often involving fluid circulation, in sedimentary rock formations. Typical examples include opal, some turquoise, and malachite. While synthetic versions of these sedimentary gems have been produced, most of the synthetic gems available on the market are single-crystal laboratory-grown versions of magmatic and metamorphic gemstones, which will be the focus of this installment.
PROCESSES FOR GROWING GEM MATERIALS IN THE LABORATORY
Some of the first crystal growth experiments involved the production of salt through evaporation of seawater. Despite some intuitive understanding of crystallization processes that enabled this advancement, the technical framework for crystal growth did not come about until more recently. An important advancement in the scientific understanding of crystal growth came with the French mineralogist Haüy, who in 1801 observed the flat cleavage planes of a broken calcite specimen. Haüy developed the idea of the “molecule intégrante” (integrant molecule) as the tiny fundamental building block of a crystal, a concept modern mineralogists recognize as the “unit cell” of a crystal (Haüy, 1801; Authier, 2013). Other natural scientists such as Steno (Winter, 1916, p. 171), Lowitz (Bohm, 1985), and Gibbs (1878) recognized, for instance, that crystal growth occurs through the attachment of material from outside the crystal, in many cases from a supersaturated solution containing the necessary chemical components.
The earliest foray into laboratory-grown gemstones was in 1837, when Gaudin grew the first small ruby crystals by mixing potassium alum, potassium chromate, lead oxide, and silica, then melting the mixture with rubies forming by vapor recrystallization (Gaudin, 1837). In the late 1800s, Verneuil succeeded in growing much larger crystals (known as boules) of flame-fusion rubies, as detailed in the next section. From there, and with further advances in crystal growth science and technology and the widespread use of synthetic crystals for many applications, the field of laboratory-grown crystals that could be used as gemstones rapidly expanded. The following sections will describe the three main synthesis techniques: melt growth, flux growth, and hydrothermal growth.
Melt Growth. Perhaps the first true laboratory-grown gemstones observed were the “Geneva” rubies first examined by Jannettaz in 1886. In an exceptional instance of gemological insight, he concluded these stones to be synthetic based on the presence of spherical gas bubbles (Jannettaz, 1886). He was able to make this logical jump, despite never having seen synthetic gemstones, by comparison with manufactured glasses and inclusions in natural rubies. The “Geneva” rubies were grown by a melt method wherein pure aluminum oxide with added chromium oxide would have been brought beyond the melting point of corundum to melt the starting material with an oxygen-hydrogen torch, allowing it to recrystallize upon cooling.
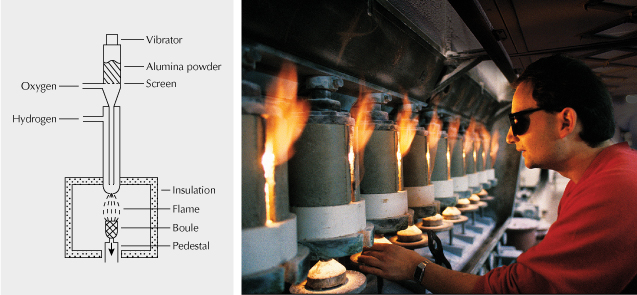
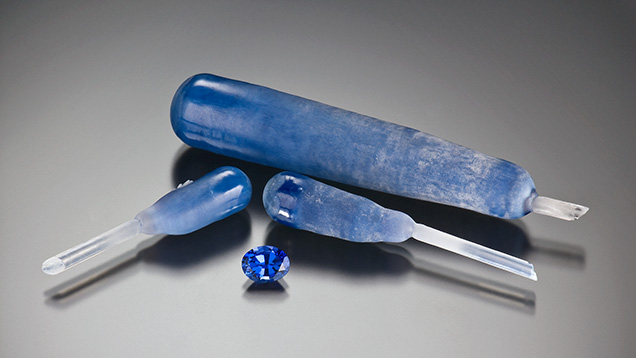
The true breakthrough, however, came in the latter part of the nineteenth century, when Verneuil perfected the flame-fusion method to produce synthetic ruby on a commercial scale (Verneuil, 1904; Nassau, 1972). His method utilized an oxygen-hydrogen torch to melt high-purity alumina powder mixed with chromium oxide that was being fed into the flame by a vibrating feeder. As the powdered starting material passes through, the flames turn the powder into small melt droplets. These melt droplets fall onto a pedestal where the temperature is lower, and the melt slowly crystallizes into a blob. As more of the alumina/chromium material is fed through the flame, the pedestal is slowly lowered, creating room for more ruby to grow, allowing the boule to elongate, and bringing it to lower and lower temperatures in a controlled manner (figure 3). This method creates a single crystal of corundum in the form of a cylindrical boule up to several inches in length (figure 4). The Verneuil crystal growth method (also known as flame-fusion growth) is the most common method for laboratory-grown ruby and sapphire to this day, accounting for the bulk of modern production. (Note that the “Geneva” rubies mentioned above are now believed to have been produced by the Verneuil process.)
While large, well-crystallized gem corundum can be synthesized with the flame-fusion method, the growth conditions are quite different from those required to produce melt-related gems naturally (i.e., magmatic gemstones). In nature, magmatic corundum generally grows around 700°–900°C, much lower than the melting point of pure corundum of 2044°C required for flame-fusion growth. The reason for this is best summarized by a quote from GIA’s John Koivula: “Nature plays in a dirty kitchen.” In other words, the high melting point only applies in a laboratory setting with pure Al2O3. Where formation occurs in the earth, the chemical environment is full of impurities that lower the melting point of corundum. This melting point depression is similar to the familiar practice of adding salt to icy sidewalks in winter months to bring down the melting point of the ice, allowing it to melt at temperatures below 0°C. The other significant difference is the pressure of formation. Flame-fusion ruby and sapphire grow at ambient pressure, whereas natural magmatic ruby and sapphire grow at higher pressures deep within the earth.
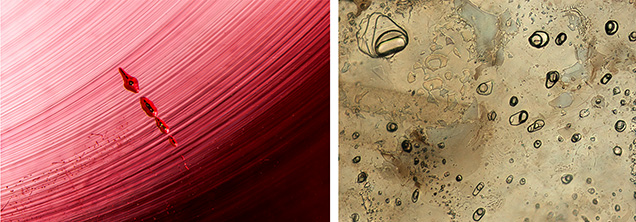
Because of these significant differences in growth conditions, the properties of flame-fusion synthetics can generally be distinguished from those of their natural counterparts. The fact that flame-fusion synthesis happens at low pressure allows gas bubbles to be captured, which is not seen in magmatic gems formed at high pressure in the absence of a free gas phase (figure 5). The flame-fusion method also produces curved striae or curved color banding, a feature not found in natural gems where growth zoning is more angular and reflects slow and deliberate crystal growth. Flame-fusion ruby and sapphire also have so-called Plato lines, observed in immersion in cross-polarized light when looking down the optic axis. Inclusions found in natural magmatic (melt-related) gemstones are entirely different and may include natural inclusions like silk or angular particulate clouds, especially in sapphires, composed of a quenched glassy melt phase and a contraction bubble (figure 5). Finally, because chemical impurities will prevent the growth of high-quality flame-fusion crystals, producers only use very pure starting materials, resulting in crystals with a very pure trace element chemistry. On the other hand, natural rubies and sapphires grow in a “dirtier” chemical environment where the corundum can take in multiple different trace elements. Flame-fusion rubies then, typically have no detectable magnesium, iron, or gallium, all components that are readily detectable in natural ruby and sapphire using only X-ray fluorescence (XRF) spectrometry. Because the composition of the melt must exactly match that of the grown crystal, the compositions of melt-grown crystals tend to be relatively simple, and gem materials with a complex chemical formula are not generally grown with melt methods.
The melting process has been further refined to include the Czochralski method (Czochralski, 1918; Nassau, 1980) and the skull method (Nassau, 1977, 1980; Harrison and Honig, 1981). In the Czochralski method, high-purity starting material is melted in a large crucible typically made of iridium. A long rotating seed crystal is then placed in contact with the melt and slowly extracted, with melt continuously crystallizing on this interface, to form a large cylindrical crystal known as a boule. The skull method passes a flame back and forth over a charge loaded into a roughly cylindrical shape and slowly melts it into a single crystal. Czochralski melt-grown oxide crystals can be quite large, potentially up to 200 mm (approximately 8 inches) in diameter and up to 600 mm (about 2 feet) in length. There are several other direct melt crystal growth methods, but the ones described here are the most significant to the synthetic gem industry. However, there are some materials that cannot be grown directly from the melt, as they do not melt congruently1. In these cases, such as with beryl, the flux and hydrothermal methods described below need to be used.
1Incongruent melting refers to situations where a mineral does not have a sharp melting point but instead disintegrates into other materials upon heating, thus making it impossible to melt and refreeze a crystal of such a mineral.
While the flame-fusion process was developed for ruby and then blue sapphire, it is now used to grow other materials such as spinel, rutile, and strontium titanate. Czochralski-grown gems include ruby and sapphire, chrysoberyl/alexandrite, spinel, garnets including YAG, and a multitude of other materials. This method is less common than flame fusion for most gem materials. The floating zone method is another melt-related technique used for gem synthesis but will not be covered here.
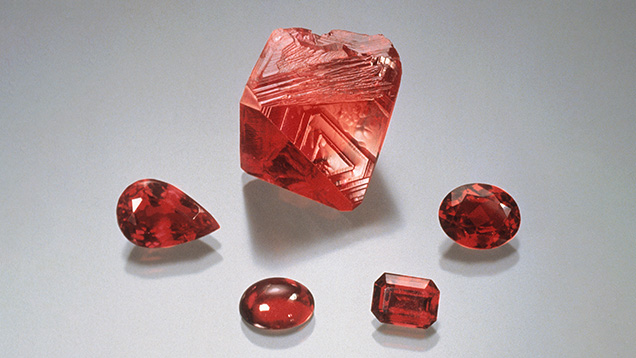
Flux Growth. Flux-grown gems (figure 6) represent another step toward more closely replicating natural geological growth conditions. As discussed above, early natural scientists began to understand gem formation as a gradual nucleation and growth of crystals by removing essential chemical components from a solution or melt. In nature, the solutions are generally either water-based hydrothermal fluids that dissolve an assortment of chemical components, including those needed to grow gems, or they are molten rocks (magmas) that contain the nutrients needed for gem growth. The flux method approaches these conditions through the use of solvents known as fluxes that are effective at dissolving the chemical components of certain gem materials at temperatures lower than those used for melt methods (i.e., the flux solution and the final crystal differ in composition) (Nassau, 1980; Elwell, 1989). Through careful control of temperature, these chemical components can nucleate to grow gem-quality crystals. The use of a flux allows the crystallization process to occur at a much lower temperature than the melting point of the gem material being grown. Additionally, flux-growth methods make it possible to grow certain gem materials that melt incongruently and therefore cannot be grown directly from a melt (e.g., beryl).
Flux-grown gem materials were first developed between 1920 and 1940 but entered the market significantly in the 1970s. However, the difficulty and expense of this method have made these materials increasingly uncommon today. Flux-grown rubies have been produced by several makers, including Chatham (Schmetzer, 1986), Douros (Smith and Bosshart, 1993), Kashan (Schmetzer, 1986), Knischka (Knischka and Gübelin, 1980), Lechleitner (Schmetzer, 1986), and Ramaura (Kane, 1983). Chemical oxide components used in the fluxes are diverse: Li2O, MoO3, PbF2, PbOx, Na3AlF6, WO3, Na2W2O7, Ta2O5, and Bi2O3, among others (Muhlmeister et al., 1998). The flux method has also been used to grow gem-quality synthetic emerald, alexandrite, sapphire, spinel, and other materials. Flux-grown crystals are generally on the order of a few millimeters up to a few centimeters in size.
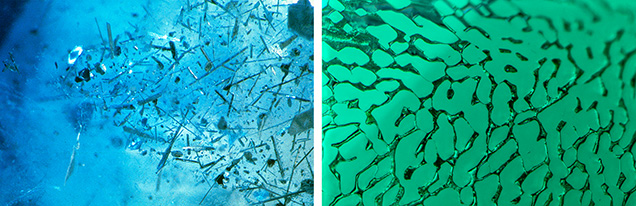
Because the growth environment is much more complex, flux-grown gemstones tend to be much more included than those produced by melt methods. Platelets of platinum or other platinum-group metals from the crucible can occur as inclusions (figure 7). These metals as well as other exotic elements can often be detected with trace element analysis using XRF or laser ablation–inductively coupled plasma–mass spectrometry (LA-ICP-MS). Their presence is considered proof of synthetic origin, as these elements are not found in any abundance in the geological environments where natural gemstones are formed.
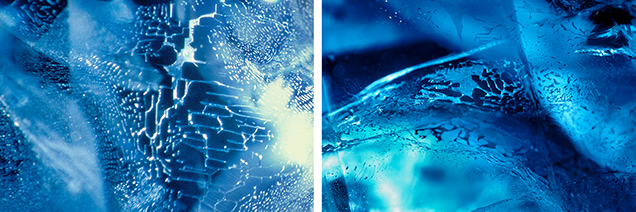
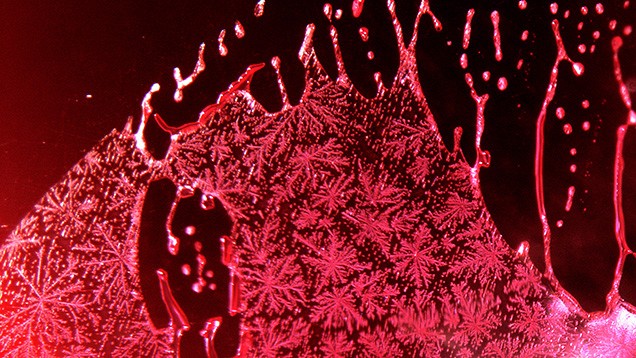
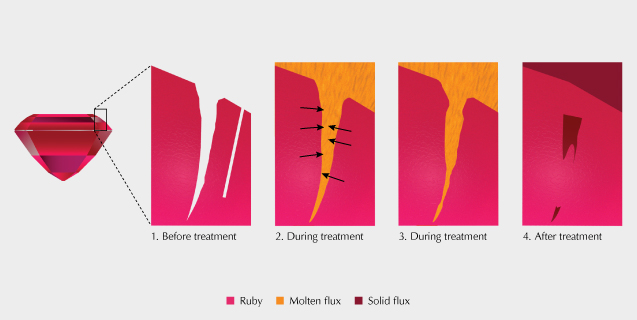
Other typical inclusions in flux-grown gemstones are flux-filled fingerprints. These are generally similar to fluid-filled fingerprints seen in natural stones. But in flux-grown synthetic gems, these fingerprints represent fractures or fissures that are filled with the liquid flux material used as a growth medium. These features have a distinct appearance and are often distinguishable from fingerprints formed in natural stones (figure 7). Fingerprints in flux-grown gems generally have a higher relief and are translucent to opaque. However, there can be some overlap in the appearance of fingerprints between flux-grown and even natural, untreated gems (figure 8). A more significant area of overlap is between flux-filled fingerprints in synthetic stones and the residue in treated, flux-healed natural stones. Many natural rubies in particular have significant fractures that detract from their clarity. Fluxes have been used during heat treatment of natural gemstones to penetrate fractures and partially fill and “heal” them, improving transparency (figure 9). After healing a fracture, the residue from a flux will remain trapped in the stone, leaving a fingerprint very similar to those seen in flux-grown gems (figure 10).
In addition to inclusion features, spectroscopy can be a useful tool for identifying some flux-grown gemstones such as synthetic alexandrite and emerald. Natural alexandrite and emerald always grow from nucleation and precipitation from some water-based hydrothermal fluid, and so these stones incorporate hydrogen derived from this water in their crystal structure. The fluxes used to grow synthetic alexandrite and emerald, on the other hand, are entirely dry (no water present). Fourier-transform infrared (FTIR) spectroscopy offers an excellent tool for detecting hydrogen species in gem materials. Natural emeralds show an intense absorption band at about 3640 cm–1 related to water in the beryl channels (figure 11, top), and natural alexandrite (figure 12, top) will show hydrogen-related peaks at 2163, 2430, and 4150 cm–1 (Malsy and Armbruster, 2012). Flux-grown emerald (figure 11, bottom) and alexandrite (figure 12, bottom) have none of these features and generally show a flat-line FTIR spectrum.
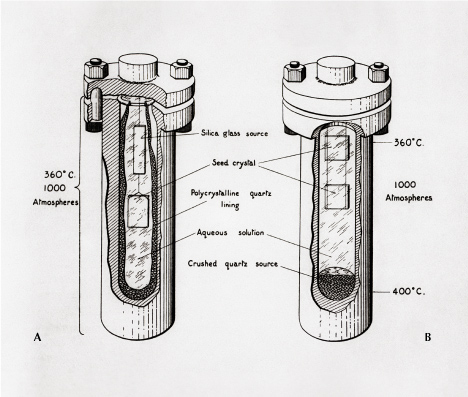
Hydrothermal Growth. Because many natural gemstones form through the action of hydrothermal fluids circulating through the earth, hydrothermally grown synthetic gemstones often pose a significant identification challenge. The first instance of hydrothermal crystal growth was by Robert Wilhelm Bunsen in 1839, when he grew crystals of barium and strontium carbonate in thick-walled glass tubes at >200°C and >100 bars (Bunsen, 1848; see also de Sénarmont, 1850). This field of research expanded, especially through efforts in the early 1900s by geologists and geochemists at the Carnegie Institution for Science and later at Harvard University (Bohm, 1985) in their attempts to understand phase equilibria in high-pressure, high-temperature environments in the earth. Use of the hydrothermal method grew rapidly from the need for high-purity quartz crystals for their piezoelectric properties, especially for radio components during World War II (McWhan, 2012). This important industrial product was historically mined from certain deposits known to yield high-quality, low-defect quartz crystals, such those in Brazil. Creating the same product artificially required hydrothermal growth (figure 13). Quartz crystals cannot be grown by melt methods, as the resulting melt is so viscous and the diffusion so slow that molten silica will quench to a glass upon cooling before crystallizing.
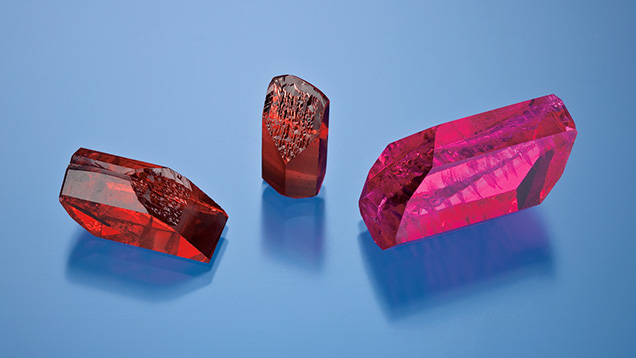
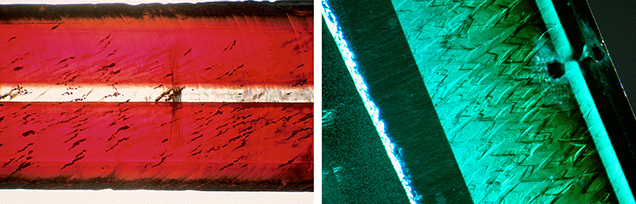
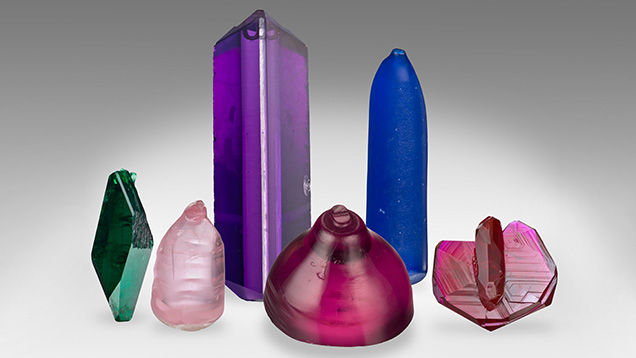
Hydrothermal growth takes advantage of the fact that water becomes a supercritical fluid at the conditions used in high-pressure autoclaves, enhancing diffusion by up to two orders of magnitude and allowing large crystals to grow. Hydrothermal growth of quartz and other gem minerals (figure 14) often uses mineralizers, salts, or other ions that increase the solubility of the gem material being grown. In quartz growth, sodium hydroxide is added to the water in the autoclave. Temperature gradients in the autoclave allow supersaturation to be achieved in the fluid, facilitating crystal growth. For industrial use, large crystals with controlled crystallographic orientation are grown using single-crystal seed plates (figure 15). In addition to quartz, hydrothermal growth methods can produce synthetic emerald (e.g., figure 16), ruby, sapphire, and spinel, among other gems.
While the conditions of hydrothermal gem synthesis often closely mimic those found in natural growth settings, most hydrothermal gems can be easily identified by an experienced gemologist. Characteristic inclusions in hydrothermal gems often include so-called chevron graining (figure 15), especially in emerald, but also features such as nailhead spicules and mineral inclusions not found in nature such as phenakite in emerald. One of the most challenging identifications is that of hydrothermal quartz. Both natural and hydrothermal synthetic quartz tend to be quite clean, meaning there are few inclusions to identify their origin. In some cases, FTIR spectroscopy can be helpful, but water-based peaks tend to be poorly resolved and FTIR spectroscopy may not always offer a conclusive identification. Observation of a seed plate can help determine hydrothermal synthetic origin, but any remnants of the seed plate are usually cut away from finished gems. Natural amethyst of sufficient size will almost always have characteristic Brazil-law twinning, which is essentially never found in hydrothermal synthetic amethyst (except in rare cases where a Brazil-law twinned seed plate is used). Trace element chemistry can sometimes prove natural origin if sufficient gallium is present, but most natural and hydrothermal synthetic quartz is extremely pure silica (SiO2) with few trace elements. As a result, chemical analysis often fails to distinguish between the two.
Synthetic Growth Processes Replicating Sedimentary Processes. Methods have also been developed to artificially grow gems that ordinarily form via sedimentary processes in the earth, such as opal, turquoise, and malachite. Of these, opal is probably the most important commercially. While the specifics of the growth methods are not fully disclosed, some solution-based method is used to dissolve SiO2 and precipitate a nanostructured lattice approximating the natural opal structure that is capable of producing play-of-color. The appearance of synthetic opal can be easily distinguished (Renfro et al., 2019). While synthetic opal matches many of the gemological properties of natural opal, there is a debate about whether it is more accurately classified as “imitation opal” (Schmetzer, 1984; Schmetzer and Henn, 1987).
CONCLUSIONS
Scientific progress in understanding crystal growth and gem formation through geological processes has been a cornerstone in humankind’s attempts to artificially grow gemstones in the laboratory. Early growth methods were sufficiently separated from nature to allow easy identification of these synthetic stones through basic observations of their inclusions and other properties. As synthesis methods became more sophisticated over time, growth conditions came to more closely approximate natural geological conditions of gem formation. An overview of different growth methods for various gem species is shown in table 1. Even with these significant technological advancements, humans have yet to fully replicate the rare conditions in the earth that produced fine natural gemstones.